ABBREVIATIONS
ABTS, 2,2′-azinobis (3-ethylbenzothiazoline 6-sulfonate); Ace, Acetone; AMPK, AMP-activated protein kinase; BHA, Butylhydroxy-anisole; BHT, Butylhydroxytoluene; CAA, Cellular antioxidant activity; COX-2, Cyclooxygenase-2; DW, Dry weight; DCM, Dichloromethane; DPPH•, 2,2-Diphenyl-1-picrylhydrazyl radical; DSS, Dextran sulfate sodium; EC50, Half maximal effective concentration; EtOH, Ethanol; FRAP, Ferric reducing antioxidant power; FW, Fresh weight; GC–QTOF-MS, Gas chromatography with quadrupole-time-of-flight mass spectrometry; GLUT4, Glucose transporter 4; GM-CSF, Granulocyte-macrophage colony-stimulating factor; GRO, Growth-regulated oncogene; HbA1c, Glycosylated hemoglobin levels; HepG2, Hepatocellular carcinoma; HPLC, High-performance liquid chromatography; HPLC-DAD-ESI-QTOF-MS, HPLC-DAD coupled to electrospray ionization quadrupole-time-of-flight mass spectrometry; HPLC-DAD-MS, HPLC-DAD with mass spectrometry; HPTLC, High-performance thin layer chromatography; HSP, Hansen’s solubility parameters; IC50, Half-maximal inhibitory concentration; iNOS, inudcible nitric oxide synthase; IL-6, Interleukin-6; IL-8, Interleukin-8; ISAPP, International Scientific Association of Probiotics and Prebiotics; LC-QTOF-MS/MS, Liquid chromatography coupled to quadrupole-time-of-flight tandem mass spectrometry; MCP-1, Monocyte chemoattractant protein-1; MCS, Mangiferin calcium salt; MeOH, Methanol; NAFLD, Non-alcoholic fatty liver disease; NF-κB, Nuclear factor κB; ORAC, Oxygen radical absorbance capacity; PAI-1, Plasminogen Activator Inhibitor-1; PLE, Pressurized liquid extraction; RP-HPLC-ESI-MS, Reversed-phase HPLC coupled to electrospray ionization mass spectrometry; SCCAI, Simple clinical colitis activity index; SCFAs, Short-chain fatty acids; TNF-α, Tumor necrosis factor-α; UHPLC, Ultra high performance liquid chromatography; UPLC-DAD-ESI-MS, UHPLC with diode array detector coupled to electrospray ionisation mass spectrometry; UPLC-ESI-QTOF-MS, UPLC coupled to electrospray ionization quadrupole-time-of-flight mass spectrometry.
INTRODUCTION
The mango (Mangifera indica L.), belonging to the Anacardiaceae family, comprises 70 genera and around 1000 varieties that differ in the fruit size, shape, color, and composition. This is one of the most important tropical fruit crops in the world [Alañón et al., 2021a; Ediriweera et al., 2017; Nguyen et al., 2022]. According to the Food and Agriculture Organization (FAO) of the United Nations, the leading tropical fruits in 2019 include pineapple (41%), avocado (29%), and mango (26%), with a trade of 7.8 million tons worldwide. Still, the tropical product with the most significant expansion is mango and its export production for 2019 was 2,042,125 tons worldwide [FAO, 2020]. Mango consumption trend is related to its sensory, nutritional, and functional qualities [Ballesteros-Vivas et al., 2019a; Borrás-Enríquez et al., 2021; Lim et al., 2019]. Many varieties or cultivars from Thailand, Mexico, Egypt, the tropical coasts of Spain, North India, Ecuador, China, and Colombia provide good-quality fruits of industrial importance, among which there are Mango Hindi [Abdel-Aty et al., 2018], Keitt [Abbasi et al., 2017; Alañón et al., 2021a, b; Kim et al., 2018], Kent [Alañón et al., 2021a, b; Marcillo-Parra et al., 2021], Osteen [Alañón et al., 2021a, b; López-Cobo et al., 2017], Halden [Castañeda-Valbuena et al., 2021; Marcillo-Parra et al., 2021], Hilacha [Hoyos-Arbeláez et al., 2018], Bombay Green, Dashe Hari, Langra and Chausa [Jyotshna et al., 2015], Sensation [Hu et al., 2018; López-Cobo et al., 2017], Xiangya [Hu et al., 2018], Tommy Atkins [Castro-Vargas et al., 2019; Marcillo-Parra et al., 2021], Luzon, Narcissus, Royal Mango, Big Tainong, Australian Mango, Thai Mango, Samall Tainong and Egg Mango [Abbasi et al., 2017], Manila [Gómez-Maldonado et al., 2020; Soria-Lara et al., 2020], Manililla [Borrás-Enríquez et al., 2021], and Ataulfo [Anaya-Loyola et al., 2020; De Ancos et al., 2018; Hernández-Maldonado et al., 2019].
The industrial processing of mangoes entails mainly extracting juices and producing nectars, concentrates, sauces, or jams with a high nutritional value [Anaya-Loyola et al., 2020; Castañeda-Valbuena et al., 2021; Sáyago-Ayerdi et al., 2019]. However, 30–60% of residues are generated during processing, including peel (12–20%) and seed (10–25%). Overall, the seed accounts for 20 to 60% of the whole fruit, as shown in Figure 1 [Lim et al., 2019; Mwaurah et al., 2020; Sánchez-Camargo et al., 2021; Torres-León et al., 2017]. Unfortunately, around 20 million tons of these residues are discarded per year, promoting water and air pollution, vegetation damage, and greenhouse gas emissions [Castañeda-Valbuena et al., 2021; Mutua et al., 2017; Sáyago-Ayerdi et al., 2019]. Nonetheless, agro-industrial by-products, such as mango peels and seeds, are a source of nutrients and bioactive compounds. For instance, the mango seed contains carbohydrates (58–80 g/100 g), proteins (6–13 g/100 g) with high levels of essential amino acids, and lipids (6–16 g/100 g) with oleic and stearic acids [Borrás-Enríquez et al., 2021; Lebaka et al., 2021; Torres-León et al., 2016]. On the other hand, mango phenolic compounds are one of the most important biologically active components [Ballesteros-Vivas et al., 2019a; Torres-León et al., 2021]. Phenolic compounds have recently gained more interest due to their beneficial health properties as antioxidants and antimicrobials agents. Several studies have reported that some compounds in mango (found in peel and seed kernel) can prevent diseases related to oxidative stress, such as cancer, arthritis, atherosclerosis, type 2 diabetes, cardiovascular diseases as well as Alzheimer’s and Parkinson’s diseases [Kim et al., 2018; Lin et al., 2020; Lopez-Martinez et al., 2022; Torres-León et al., 2021]. The high flavonoid content is widely linked to antioxidant and antiproliferative activity [Abbasi et al., 2017; Soria-Lara et al., 2020]. Additionally, the high phenolic compound content has been reported to beneficially modify the gut microbiota profile [Bento-Silva et al., 2020; Kim et al., 2018]. In this context, the mango wastes contain diverse and valuable compounds that offer uncountable benefits to human health. Therefore, the main goal of this review article was to understand the bioactive potential of phenolic compounds from mango by-products, mainly in the context of prebiotics-like actions. It also discusses extraction methods used for phenolic identification and quantification.
Figure 1
(A) Structural components of the mango fruit (M. indica L.); (B) main phenolic compounds in mango agro-industrial residues [De Ancos et al., 2018; Ediriweera et al., 2017].
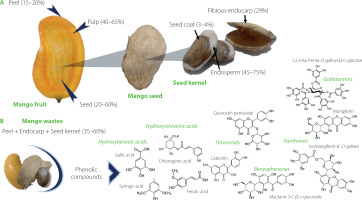
PHENOLIC COMPOUNDS IN MANGO BY-PRODUCTS
Composition, distribution, extraction, and identification
Phenolic compounds from mango residues can be classified into phenolic acids, flavonoids, gallates and gallotannins, ellagitannins, and others (benzophenones, xanthones, and lignans) [Ballesteros-Vivas et al., 2019a; De Ancos et al., 2018; Torres-León et al., 2021] as listed in Table 1. These compounds can be found in both free and bound forms [Alañón et al., 2021a; Gómez-Caravaca et al., 2016; Pacheco-Ordaz et al., 2018a]. Generally, the bound compounds are low molecular weight phenolics that may be interacting with macromolecules or cell wall components such as dietary fiber; these can be phenolic acids such as gallic, p-hydroxybenzoic, vanillic, protocatechuic, p-coumaric, ferulic, ellagic, as well as trans and cis-p-coumaric acids [Abbasi et al., 2017; Anaya-Loyola et al., 2020; Hernández-Maldonado et al., 2019]. Gómez-Caravaca et al. [2016] reported ellagic acid as the main bound phenolic in the pulp and peel fruit, as well as the husk and the seed kernel. The authors have remarked that this compound is responsible for 88.7% of the bound phenolics in the peel of mango fruit. Nonetheless, the variation of phenolic composition and biological activities in mango depends on the plant variety or cultivar, its geographic origin, growing conditions, age, fruit harvest time, degree of maturity, processing, and storage conditions [Abdel-Aty et al., 2018; Alañón et al., 2021a; Marcillo-Parra et al., 2021; Sáyago-Ayerdi et al., 2019]. For example, fisetin and mangiferin have been found in higher contents in the mango peel of the cultivar Xiao Tainong compared to other varieties [Abbasi et al., 2017]. It has also been reported that the Keitt mango peel had a significantly higher total phenolic content than the Kent and Osteen varieties; mainly due to the presence of galloyl glucose, 5-galloylquinic acid, digalloylquinic acid, hexagalloyl glucose, and galloyl glucoside [Alañón et al., 2021a]. Moreover, it was reported that green mango peels had about 45% higher content of phenolics than ripe mango. This trend has been associated with the mango ripening process because physiological, biochemical, and molecular changes develop, inducing the degradation, synthesis, or accumulation of phenolic compounds [Alañón et al., 2021b; Hu et al., 2018].
Table 1
Phenolic compounds identified in mango by-products.
Class/Subclass | Compound | Content | Extraction solvent | Analytical method | References | ||
---|---|---|---|---|---|---|---|
Peel | Seed kernel | Unit (based on DW) | |||||
Phenolic acids/Hydroxybenzoic acids and derivatives | Gallic acid | 2.30-25.62 | 2.52-26.08 | mg/100 g | 80% MeOH | HPLC-DAD-ESI-QTOF-MS | Alañón et al. [2021a, b]; Gómez-Caravaca et al. [2016] |
28.60-43.10 | nd | 70% Ace | HPLC-UV/ Vis | Marcillo-Parra et al. [2021] | |||
p-Hydroxybenzoic acid | nd | 0.54 | mg/g | 80% EtOH | HPLC | Gómez-Maldonado et al. [2020] | |
p-Hydroxybenzoic acid glucoside | 0.64-17.24 | 6.06-11.01 | mg/100 g | 80% MeOH | HPLC-DAD-ESI-QTOF-MS | Alañón et al. [2021a, b]; Gómez-Caravaca et al. [2016]; López-Cobo et al. [2017] | |
p-Hydroxybenzoic acid glucoside isomer I | 6.34-16.66 | nd | μg/100 g | López-Cobo et al. [2017] | |||
p-Hydroxybenzoic acid isomer I | 968.36-2186.12 | 835.62-2186.12 | |||||
p-Hydroxybenzoic acid isomer II | 173.97-982.15 | 729.36-982.15 | |||||
p-Hydroxybenzoic acid isomer III | nd | 259.42-846.12 | |||||
Dihydroxybenzoic acid glucoside | 0.30-3 | 1.13-4.53 | mg/100 g | Alañón et al. [2021a, b] | |||
Protocatechuic acid | 158.44-434.24 | 295.82-479.05 | μg/100 g | López-Cobo et al. [2017] | |||
Syringic acid | 7.47-24.09 | 25.44-54.47 | mg/100 g | Gómez-Caravaca et al. [2016]; López-Cobo et al. [2017] | |||
Vanillic acid | nd | 21.6 | mg/100 g | 80% EtOH | HPLC | El-Kady et al. [2017] | |
30.47-48.21 | nd | μg/100 g | 80% MeOH | HPLC-DAD-ESI-QTOF-MS | López-Cobo et al. [2017] | ||
Vanillic acid glucoside | 11.6-62.69 | 6.75-16.86 | mg/100 g | Alañón et al. [2021a, b]; López-Cobo et al. [2017] | |||
Ethyl 2,4-dihydroxy-3-(3,4,5-trihydroxybenzoyl)oxybenzoate | 7.17 | 6.29 | Gómez-Caravaca et al. [2016] | ||||
Ellagic acid | nd | 2613 | mg/100 g | 80% EtOH | HPLC | El-Kady et al. [2017] | |
0.40-1.30 | 6.66-614.89 | 0% MeOH | HPLC-DAD-ESI-QTOF-MS | Alañón et al. [2021a, b]; Gómez-Caravaca et al. [2016] | |||
Phenolic acids/Hydroxycinnamic acids and derivatives | Caffeic acid | nd | 2.04 | mg/g | 80% EtOH | HPLC | Gómez-Maldonado et al. [2020] |
Chlorogenic acid | 42.07 | nd | μg/g | 50% MeOH | Safdar et al. [2017] | ||
Chlorogenic acid | nd | 8.50 | mg/100 g | 80% MeOH | Abdel-Aty et al. [2018] | ||
p-Coumaric acid | nd | 12.50 | Abdel-Aty et al. [2018] | ||||
trans-p-Coumaric acid | 107.88-1081.61 | 107.88-155.55 | μg/100 g | 80% MeOH | HPLC-DAD-ESI-QTOF-MS | Alañón et al. [2021a, b]; López-Cobo et al. [2017] | |
cis-p-Coumaric acid | 752.87-861.14 | nd | |||||
p-Coumaric acid glucoside | 0.30-11.58 | 1.51 | mg/100 g | ||||
Ferulic acid | 80.58-382.81 | 341.11-382.81 | μg/100 g | López-Cobo et al. [2017] | |||
Ferulic acid hexoside | 1.20-2.10 | nd | mg/100 g | Alañón et al. [2021a, b] | |||
Sinapic acid | nd | 17.63 | 80% EtOH | HPLC | El-Kady et al. [2017] | ||
Sinapic acid hexoside | 0.80-1.10 | nd | mg/100 g | 80% MeOH | HPLC-DAD-ESI-QTOF-MS | Alañón et al. [2021a, b]; Gómez-Caravaca et al. [2016]; López-Cobo et al. [2017] | |
Sinapic acid hexoside-pentoside | 10.40-50.99 | nd | |||||
Dihydro sinapic acid hexoside-pentoside | 4.80-62.59 | nd | |||||
Flavonoids/Flavanones | Eriodictyol | nd | 2.98-5.35 | mg/100 g | 80% MeOH | HPLC-DAD-ESI-QTOF-MS | López-Cobo et al. [2017] |
Hesperidin | nd | 3000 | HPLC | Abdel-Aty et al. [2018] | |||
Flavonoids/Flavanols | Catechin | 0.03-0.25 | nd | mg/100 g | 80% Ace | HPLC | Abbasi et al. [2017] |
88.50 | nd | μg/g | 50% EtOH | Safdar et al. [2017] | |||
6.37-27.87 | 3.57-25.37 | mg/100 g | 80% MeOH | HPLC-DAD-ESI-QTOF-MS | Alañón et al. [2021a, b]; Gómez-Caravaca et al. [2016] | ||
Epicatechin | 9.01-9.24 | nd | mg/100 g | 70% Ace | HPLC-UV/ Vis | Marcillo-Parra et al. [2021] | |
Flavonoids/Flavonols | Fisetin | 1.86-17.49 | nd | mg/100 g | 80% Ace | HPLC | Abbasi et al. [2017] |
Kaempferol | nd | 4 | 80% EtOH | El-Kady et al. [2017] | |||
Kaempferol | 31.73 | nd | μg/g | 50% EtOH | Safdar et al. [2017] | ||
Myricetin | 7.05 | nd | |||||
Myricetin | nd | 4.92 | mg/g | 80% EtOH | Gómez-Maldonado et al. [2020] | ||
Quercetin | 35.20 | nd | μg/g | 50% MeOH | Safdar et al. [2017] | ||
nd | 3.87 | mg/g | 80% EtOH | Gómez-Maldonado et al. [2020] | |||
2.05-3.32 | nd | mg/100 g | 70% Ace | HPLC-UV/ Vis | Marcillo-Parra et al. [2021] | ||
Quercetin arabinofuranoside | 4.19-19.41 | nd | mg/100 g | 80% MeOH | HPLC-DAD-ESI-QTOF-MS | Alañón et al. [2021a, b]; Gómez-Caravaca et al. [2016]; López-Cobo et al. [2017] | |
Quercetin hexoside | 1.43 | nd | g/kg | 50% EtOH | HPLC-DAD | De Ancos et al. [2018] | |
Quercetin pentoside | 1.72 | nd | |||||
Quercetin glucoside | 19.50-188.69 | 1.02-2.26 | mg/100 g | 80% MeOH | HPLC-DAD-ESI-QTOF-MS | Alañón et al. [2021a, b]; López-Cobo et al. [2017] | |
Quercetin galactoside | 10.90-120.17 | 0.18 | |||||
Quercetin pentoside | 1.72 | nd | |||||
Quercetin xyloside | 2.70-39.18 | nd | |||||
Rhamnetin hexoside | 5.22-28.03 | nd | López-Cobo et al. [2017] | ||||
Rutin | 98.43 | nd | μg/g | 50% MeOH | HPLC | Safdar et al. [2017] | |
nd | 2.95 | mg/g | 80% EtOH | Gómez-Maldonado et al. [2020] | |||
26.60-46.50 | nd | mg/100 g | 70% Ace | HPLC-UV/ Vis | Marcillo-Parra et al. [2021] | ||
Apigenin | nd | 0.53 | mg/g | 80% EtOH | HPLC | Gómez-Maldonado et al. [2020] | |
Flavonoids/Anthocyanins | Cyanidin 3-O-β-d-galactopyranoside | 0.138-1.108 | nd | mg/100 g | 80% MeOH | HPLC-DAD-ESI-QTOF-MS | López-Cobo et al. [2017] |
7-O-Methylcyanidin 3-O-β-d-galactopyranoside | 0.58-3.63 | nd | |||||
Petunidin rutinoside-(p-coumaric acid) gallate | 0.38-0.59 | 0.37-0.46 | |||||
Gallotannins and gallic acid derivatives | Tannic acid | nd | 987 | mg/100 g | 80% MeOH | HPLC | Abdel-Aty et al. [2018] |
Coumaroyl galloyl glucoside | 2.34-19.50 | nd | HPLC-DAD-ESI-QTOF-MS | Alañón et al. [2021a, b]; Gómez-Caravaca et al. [2016]; López-Cobo et al. [2017] | |||
Coumaroyl galloyl glucoside isomer I | 4.35-4.43 | nd | López-Cobo et al. [2017] | ||||
Coumaroyl galloyl glucoside pentoside | 0.80-2.24 | nd | Alañón et al. [2021a]; López-Cobo et al. [2017] | ||||
Coumaroyl galloyl glucoside pentoside isomer I | 1.85-1.99 | nd | López-Cobo et al. [2017] | ||||
Galloyl glucoside | 0.44-109.60 | 154-271 | Alañón et al. [2021a, b]; López-Cobo et al. [2017] | ||||
Galloyl glucoside isomer I | 59.88-372.97 | 63.10-101.36 | mg/100 g | 80% MeOH | HPLC-DAD-ESI-QTOF-MS | López-Cobo et al. [2017] | |
Galloyl glucoside isomer II | 7.56-14.13 | nd | |||||
Galloyl glucoside isomer III | 3.09-5.03 | nd | |||||
Galloyl glucoside isomer IV | 2.53-4.09 | nd | |||||
Galloylglucose isomer V | 1.80-3.78 | nd | |||||
Galloyl glucose isomer VI | 18.91-29.64 | nd | |||||
Galloyl diglucoside | 3.80-51.81 | 2.54-11.28 | Alañón et al. [2021a, b] | ||||
Galloyl diglucoside isomer II | 1.90-3.90 | nd | López-Cobo et al. [2017] | ||||
Galloyl diglucoside isomer III | 8-16 | 1.43-5.19 | |||||
Galloyl diglucoside isomer IV | 28.67-63.26 | 6.33-13.44 | mg/100 g | 80% MeOH | HPLC-DAD-ESI-QTOF-MS | Gómez-Caravaca et al. [2016]; López-Cobo et al. [2017] | |
3-Galloylquinic acid | 1-3 | 1.95-2.78 | Alañón et al. [2021a, b] | ||||
5-Galloylquinic acid | 0.20-200.20 | 3.57-84.46 | Alañón et al. [2021a, b]; López-Cobo et al. [2017] | ||||
7-O-Galloyltricetiflavan | 0.10-2.80 | 4.90-13.99 | Alañón et al. [2021a, b]; Gómez-Caravaca et al. [2016] | ||||
Digallic acid | 0.70-10.83 | 1.04-3.64 | Alañón et al. [2021a, b]; Gómez-Caravaca et al. [2016] | ||||
m-Digallic acid methyl ester | 2.68 | nd | g/kg | 50% EtOH | HPLC-DAD | De Ancos et al. [2018] | |
Digalloyl glucose | 20.10-47.28 | 0.70-4.23 | mg/100 g | 80% MeOH | HPLC-DAD-ESI-QTOF-MS | Alañón et al. [2021a, b]; Gómez-Caravaca et al. [2016] | |
Digalloyl glucose isomer I | 0.63-3.21 | nd | López-Cobo et al. [2017] | ||||
Digalloylquinic acid | 0.50-74.20 | 1.11 | Alañón et al. [2021a, b] | ||||
3,5-Digalloylquinic acid | 2.69 | nd | López-Cobo et al. [2017] | ||||
5-(Digalloyl)quinic acid | 0.34-10.12 | nd | |||||
Ethyl 2,4-dihydroxy-3-(3,4,5-trihydroxybenzoyl) oxy)-benzoate | 7.17 | 5.85-11.30 | |||||
Trigalloyl glucose | 6.1-17.70 | 0.44-3.15 | Alañón et al. [2021a, b]; Gómez-Caravaca et al. [2016] | ||||
Trigalloyl glucose isomer I | 1.22-1.53 | nd | López-Cobo et al. [2017] | ||||
Tetragalloyl glucose | 3.4-11.6 | 23.21-68.98 | Alañón et al. [2021a, b] | ||||
Tetragalloyl glucose isomer I | 1.87-4.71 | 1.04-3.52 | López-Cobo et al. [2017] | ||||
Tetragalloyl glucose isomer II | 4.54-6.37 | 2.14-6.78 | |||||
Tetragalloyl glucose isomer III | 1.12-2.37 | 0.46-0.67 | |||||
Pentagalloyl glucose | 24.02-36.63 | 92.60-207.41 | |||||
Hexagalloylglucose | 21.03-73.10 | 61.67-172.99 | Alañón et al. [2021a, b]; Gómez-Caravaca et al. [2016] | ||||
Hexagalloylglucose isomer I | 17.44-73.81 | 10.00-46.56 | López-Cobo et al. [2017] | ||||
Hexagalloylglucose isomer II | 11.74-36.26 | 7.98-29.91 | |||||
Hexagalloylglucose isomer III | 3.33-27.70 | 0.98-15.54 | |||||
Hexagalloylglucose isomer IV | 0.46-4.60 | 0.54-10.25 | |||||
Heptagalloyl glucose | 35.10-57.8 | 6.15 | Alañón et al. [2021a, b] | ||||
Heptagalloylglucose isomer III | 3.71-35.04 | nd | López-Cobo et al. [2017] | ||||
Heptagalloylglucose isomer IV | 7.35-48.18 | 0.59-2.32 | |||||
Heptagalloylglucose isomer V | 7.80-44.86 | 2.03-5.24 | |||||
Heptagalloylglucose isomer VI | 5.27-28.43 | 0.05-1.03 | |||||
Heptagalloylglucose isomer VII | 4.56-23.33 | 0.02-0.51 | |||||
Heptagalloylglucose isomer VIII | 1.38-7.38 | nd | |||||
Hydroxybenzoyl galloyl glucoside | 0.58-2.22 | nd | |||||
Ethyl gallate | 1.38 | nd | g/kg | 50% EtOH | HPLC-DAD | De Ancos et al. [2018] | |
Methyl gallate | 51.10-167.60 | 94.43-558.86 | mg/100 g | 80% MeOH | HPLC-DAD-ESI-QTOF-MS | Alañón et al. [2021a, b]; López-Cobo et al. [2017]; | |
Methyl digallate ester | 46.73-66.55 | 19.61-47.65 | López-Cobo et al. [2017] | ||||
Benzophenones | Iriflophenone glucoside | nd | 0.61-10.89 | mg/100 g | 80% MeOH | HPLC-DAD-ESI-QTOF-MS | Alañón et al. [2021b]; López-Cobo et al. [2017] |
Maclurin C-glucoside | nd | 4.38-11.40 | Alañón et al. [2021b]; Gómez-Caravaca et al. [2016] | ||||
Maclurin C-glucoside isomer I | 1.05-3.64 | 4.17-11.77 | Gómez-Caravaca et al. [2016]; López-Cobo et al. [2017] | ||||
Maclurin-3-C-β-d-glucoside | 0.42 | nd | g/kg | 50% EtOH | HPLC-DAD | De Ancos et al. [2018] | |
Maclurin-3-C-(2-O-galloyl)- β-d-glucoside | 1.59 | nd | |||||
Maclurin-3-C-(2,3-di-O-galloyl)-β-d-glucoside | 1.33 | nd | |||||
Maclurin-3-C-(6′-p-hydroxybenzoyl glucoside | 1.21 | nd | |||||
Maclurin galloyl glucoside | 2.36-4.73 | 0.07-9.63 | mg/100 g | 80% MeOH | HPLC-DAD-ESI-QTOF-MS | Alañón et al. [2021a, b]; Gómez-Caravaca et al. [2016]; López-Cobo et al. [2017] | |
Maclurin digalloyl glucoside | 0.30-1.89 | 0.47-6.21 | |||||
Xanthones | Lupeol | 0.05-0.30 | nd | mg/100 g | EtOH-Ace (7:3, v/v) | HPTLC | Jyotshna et al. [2015] |
Mangiferin | 4.14-29.78 | 22.48-148.12 | mg/100 g | 80% MeOH | HPLC-DAD-ESI-QTOF-MS | Alañón et al. [2021a, b]; Gómez-Caravaca et al. [2016]; López-Cobo et al. [2017] | |
Mangiferin-6´-O-gallate | 1.57 | nd | g/kg | 50% EtOH | HPLC-DAD | De Ancos et al. [2018] | |
Mangiferin-3-C-(2,3-di-O-galloyl)-β-d-glucoside | 1.44 | nd | |||||
Isomangiferin-6´-O-gallate | 1.34 | nd | |||||
Methoxylmangiferin | nd | 2.24 - 4.43 | mg/100 g | 80% MeOH | HPLC-DAD-ESI-QTOF-MS | López-Cobo et al. [2017] | |
Lignans | Pentadecenylresorcinol | 3.87-16.50 | 0.53-0.96 | μg/g | DCM | GC-QTOF-MS | López-Cobo et al. [2017] |
Pentadecylresorcinol | 56.58-110.76 | 0.84-2.96 | |||||
Heptadecadienylresorcinol | 224.77-602.26 | 0.84-2.96 | |||||
Heptadecenylresorcinol | 271.40-873.99 | 3.29-14.32 | |||||
Heptadecylresorcinol | 116.9 | nd | |||||
Nonadecenylresorcinol | 4.92-44.61 | 1.14-7.74 | |||||
Others | Pyrogallol | nd | 1337.66 | mg/100 g | 80% EtOH | HPLC | El-Kady et al. [2017] |
Catechol | nd | 202 |
[i] DW, dry weight; EtOH, ethanol; MeOH, methanol; Ace, acetone; DCM, dichloromethane; nd, not detected; HPLC, high-performance liquid chromatography; HPLC-DAD, HPLC with a diodearray detector; HPLC-UV/Vis, HPLC with UV-vis detection; HPLC-DAD-ESI-QTOF-MS, HPLC-DAD coupled to electrospray ionisation-quadrupole-time-of-flight mass spectrometry (ESI-QTOF-MS); HPTLC, high-performance thin layer chromatography; GC-QTOF-MS, gas chromatography with quadrupole-time-of-flight mass spectrometry.
Nowadays, phenolics from natural sources are attracting increased attention for their bioactivities. For that reason, many studies have focused on improving extraction techniques and characterization of phenolic profiles. Due to the vast diversity of phenolic compounds, no common global strategy has been developed to carry out their extraction and identification. They differ in physical and chemical properties, and above all in solubility, which is why the efficiency of their extraction process depends mainly on the solvents and techniques used. Moreover, the source from which they are obtained determines the extraction process since phenolic compounds can interact with other components of the matrix and, as mentioned above, can be present in the tissues in free or bound form [Abbasi et al., 2017; Alañón et al., 2021a; Pacheco-Ordaz et al., 2018a]. Hence, the need arises to develop the optimum and appropriate methods for their proper extraction and purification. Essential factors for optimal extraction, such as pH, time, and temperature, have been considered [Ballesteros-Vivas et al., 2019a; Castañeda-Valbuena et al., 2021; Martínez-Ramos et al., 2020]. However, the most important aspect is the solvent type used (Table 1). The solvents used so far in the case of mango by-products included ethanol (50, 80, 96, and 100%, v/v) [Azhar et al., 2019; Buelvas-Puello et al., 2021; Gómez-Maldonado et al., 2020], methanol (50 and 100%, v/v) [Castro-Vargas et al., 2019; Hoyos-Arbeláez et al., 2018], ethyl acetate [Ballesteros-Vivas et al., 2019a; Torres-León et al., 2021], and hexane [Martínez-Ramos et al., 2020; Torres-León et al., 2021].
Numerous phenolic compounds have been identified and characterized through different approaches. For instance, Ballesteros-Vivas et al. [2019a] identified xanthones, phenolic acids, flavonoids, and gallotannins in mango seed kernels. Different contents of mangiferin were determined, depending on the solvent type and extraction method used, i.e.: 13.27 mg/g when absolute ethanol and pressurized liquid were applied; 13.60 and 12.34 mg/g when kernels were macerated in 50% (v/v) and absolute ethanol, respectively. In turn, Martínez-Ramos et al. [2020] reported that the ethanol-to-acetone ratio of the extraction mixture affected the extraction efficiency of phenolic compounds from mango peel residues during conventional and ultrasound-assisted processes. Furthermore, they showed that the composition of solvent mixtures and ultrasonic power influenced the solvent-solute interaction. On the other hand, Castañeda-Valbuena et al. [2021] established that variations in the solvent-solid ratio and solvent concentration used to obtain mango peel and seed extracts influenced their antioxidant capacity evaluated by different methods (DPPH, ABTS, and FRAP assays). The conventional and new alternative techniques deployed so far for the extraction of phenolic compounds from residues mango include: maceration [Ballesteros-Vivas et al., 2019a; Borrás-Enríquez et al., 2021; Safdar et al., 2017], agitation [El-Kady et al., 2017; Martínez-Ramos et al., 2020], Soxhlet and solvent extraction [Buelvas-Puello et al., 2021; Castro-Vargas et al., 2019; Torres-León et al., 2021], solid-liquid extraction, ultrasound-assisted extraction [Borrás-Enríquez et al., 2021; Castañeda-Valbuena et al., 2021; Lopez-Martinez et al., 2022], microwave-assisted extraction [Martínez-Ramos et al., 2020; Sánchez-Camargo et al., 2021; Torres-León et al., 2017], extraction assisted by fermentation [Torres-León et al., 2021], high hydrostatic pressure [Cádiz-Gurrea et al., 2020; Sánchez-Camargo et al., 2021], extraction with supercritical fluids [Ballesteros-Vivas et al., 2019b; Buelvas-Puello et al., 2021; Sánchez-Camargo et al., 2019], and extraction with pressurized fluids [Santana et al., 2019].
Recent trends in the valorization of agro-industrial wastes are focused on minimizing the environmental impact through the circular economy model. In this context, green techniques are essential to reduce the adverse effects on human health and the environment. Thereby, using green solvents in phenolic extraction provides more environmentally friendly and sustainable processes [Cádiz-Gurrea et al., 2020; Oliver-Simancas et al., 2021]. Ballesteros-Vivas et al. [2019a] proposed the integrated approach of green extraction, based upon sequential pressurized liquid extraction (PLE) to obtain fractions rich in bioactive compounds. The extraction of bioactive components with high antioxidant and antiproliferative capacity from residues of the mango seeds was optimized; the most efficient proved to be the mixture of ethanol/ethyl acetate (50:50, v/v) at 25°C and 1.01 bar, based on Hansen’s solubility parameters (HSP). PLE is an alternative technology to reduce environmental pollution generated by standard extraction methods. This technique allows for higher extraction yields of bioactive compounds with lower solvent consumption and shorter extraction time [Cádiz-Gurrea et al., 2020].
Regarding the identification and quantification (Table 1) of the phenolic compounds of mango residues, various methods based on liquid chromatography (HPLC, UPLC) with detection by UV-Vis detector or DAD were used [Buelvas-Puello et al., 2021; Lopez-Martinez et al., 2022; Sánchez-Camargo et al., 2019]. The techniques combining liquid chromatography with mass spectrometry were also often applied: RPHPLC-ESI-MS [Torres-León et al., 2017, 2021], HPLC-DAD-MS [Hernández-Maldonado et al., 2019], HPLC-DAD-ESI-QTOF-MS [Alañón et al., 2021a, b; Gómez-Caravaca et al., 2016; López-Cobo et al., 2017], [UPLC-DAD-ESI-MS [Navarro et al., 2019], UPLC-ESI-QTOF-MS [Anaya-Loyola et al., 2020; Hu et al., 2018], and LC-QTOF-MS/MS [Ballesteros-Vivas et al., 2019a; Sánchez-Camargo et al., 2021]. Other chromatographic techniques successfully used to identify mango bioactive compounds were HPTLC [Jyotshna et al., 2015] and GC-QTOF-MS [López-Cobo et al., 2017].
Mango agro-industrial wastes contain xanthonoids in the C-glucoside form, such as mangiferin. This polyphenol and some of its derivatives, such as methoxymangiferin and isomangiferin, have been detected in the peel and kernel residues [Alañón et al., 2021a, b; Anaya-Loyola et al., 2020; Marcillo-Parra et al., 2021]. Mangiferin has been reported to possess numerous beneficial properties, such as antioxidant, anti-inflammatory, antiviral, antiparasitic, and antimicrobial activities [Anaya-Loyola et al., 2020; Hernández-Maldonado et al., 2019; Vazquez-Olivo et al., 2019]. Mango residues also contain gallic acid, its numerous derivatives and gallotannins. Gallotannins are hydrolysable tannins with a structure of polymeric chains of gallic acid esterified with glucose. Gallotannins and their derivatives represent 20–28% of the total phenolic content in the mango peel. Overall, they are recognized as mango nutraceutical components for their pharmacological effects and functional properties [Alañón et al., 2021a]. Interestingly, the gallotannins identified in the mango seed kernels were considered as an indicator of the degree of maturation among multiple mango varieties [Alañón et al., 2021b; Torres-León et al., 2021]. Yet, another study with residues of the Ataulfo variety identified 47 phenolic compounds by UPLC-ESI-QTOF MS [Anaya-Loyola et al., 2020]. Of these, 35 were detected in the extractable fraction and 12 in the fraction after acid hydrolysis. The extractable fraction contained gallotannins, such as heptagalloyl hexose, hexagalloyl hexose, pentagalloyl hexose, tetragalloyl hexose, and some gallic acid derivatives (gallic acid hexoside, gallic acid dihexoside, and galloylquinic acid). In contrast, the fraction obtained after hydrolysis contained 59% of ellagic acid and 22.4% of gallic acid, and other phenolics, like rhamnetin, syringic acid, and vanillic acid.
Mango peel
Among the mango peel phenolic compounds identified, worthy of special attention are mangiferin, gallic acid, quercetin, hydrolysable tannins, syringic acid, ferulic acid, and protocatechuic acid [Anaya-Loyola et al., 2020; Borrás-Enríquez et al., 2021; Pacheco-Ordaz et al., 2018a; Soria-Lara et al., 2020]. A study conducted by Tan et al. [2020] identified 651 secondary metabolites in the peel of two mango varieties by the metabolome approach, of which 257 corresponded to phenolic compounds, including numerous anthocyanin proanthocyanidins, and hydrolysable tannins, as well as gallocatechin, gallocatechin gallate, epicatechin, epicatechin gallate epicatechin 3-O-gallate, protocatechuic aldehyde, 3,4-dihydroxybenzoic acid, and ellagic acid. Navarro et al. [2019] also detected various gallotannins with different degrees of polymerization, including monogalloyl hexose up to undecagalloyl hexose. A comparative study of the total phenolic content of the mango different tissues demonstrated the peels from fruits with commercial maturity had a higher content (59.75 mg gallic acid equivalent (GAE)/g) than the pulp (10.73 mg GAE/g). More than 100 phenolic compounds (free and bound) were found in the peel, including phenolic acids, benzophenones, flavonoids, and gallotannins (mono/digalloyl) [Alañón et al., 2021a]. Monogalloyl derivatives represented around 34–37% of phenolics, with galloyl glucose prevailing. Regarding gallates, the digalloyl derivatives accounted for 16–29%, with high contents of digalloyl glucose, digalloylquinic acid, digallic acid, and the digallic acid methyl ester. The flavonoid fraction (10–19%) included flavonols (quercetin and its glycosides with glucosyl, galactosyl, xylosyl, arabinopyrosyl, and arabinofurosyl moieties) and flavanols (catechin and 7-O-galloyltricetyl flavane). Phenolic acids, like vanillic, p-hydroxybenzoic, ellagic, p-coumaric, sinapic, and ferulic acid, were also found, accounting for 5–10% of the peel phenolics [Alañón et al., 2021a]. Benzophenones, including maclurin, and their derivatives have been also identified in the peel with maclurin galloyl glucoside being the most abundant. Among xanthones, mangiferin has been found to be one of the major compounds in mango peel and recognized currently as one of the strongest antioxidants [Hernández-Maldonado et al., 2019; Marcillo-Parra et al., 2021; Navarro et al., 2019; Vazquez-Olivo et al., 2019].
Mango seed kernel
Mango seed is constituted by kernel or embryo (45–75%), the shell of fibrous endocarp (29%), and seed coat (3%) (Figure 1). Although the mango seed generates a large mass of waste products, little progress has been made on the valorization of these by-products since the only recycling strategy has focused on converting waste into animal feeds. Notwithstanding, these residues are a good source of bioactive compounds. Therefore, using this waste appropriately could generate bio-products with added value [Ballesteros-Vivas et al., 2019a; Borrás-Enríquez et al., 2021; Mutua et al., 2017].
The total phenolic content of mango kernel is 28 times higher (78–80.5 mg GAE/g) than that of the pulp (2.1–4.2 mg GAE/g). Moreover, contents of some individual phenolics, as gallic acid and its derivatives, in mango kernels are higher than in other fruits’ seeds, such as longan (Dimocarpus longan Lour.) seed [Alañón et al., 2021b]. Phenolic acids (gallic, ellagic, protocatechuic, ferulic, caffeic, coumaric, cinnamic, vanillic, and 4-caffeylquinic acid), flavonoids (rhamnetin-3-(6’-2-butenoil-hexoside), quercetin, kaempferol, rutin, and anthocyanins), tannins and derivatives (ethyl galate and penta-O-galloyl glucoside), xanthones (mangiferin, isomangiferin, homomangiferin), and benzophenones were identified in the phenolic profile in several studies [Lebaka et al., 2021; Mwaurah et al., 2020; Torres-León et al., 2017]. Therein, penta-O-galloyl glucoside is an important compound linked with antimicrobial, anti-inflammatory, antiproliferative, anticancer, antidiabetic, and antioxidant properties. Likewise, phenolics have been reported with bioactivity in breast, liver, leukemia, cervix, prostate, lung, and colon cancer cell lines [Ballesteros-Vivas et al., 2019a; Castro-Vargas et al., 2019; Kim et al., 2018; Torres-León et al., 2021]. Hesperidin and compounds with monogalloyl moiety (galloyl glucose, galloyl diglucose, 5-galloylquinic acid, 3-galloylquinic acid, methyl galate, and ethyl gallate) have also been detected in high contents [Abdel-Aty et al., 2018; Alañón et al., 2021b; Ballesteros-Vivas et al., 2019a]. Derivatives of digallic acid (from gallic acid esterification), including digallic acid, digalloyl glucose, digallic acid methyl ester and digallic quinic acid have been detected as well [Alañón et al., 2021a, b; Gómez-Caravaca et al., 2016].
In the mango seed kernel, various flavan-3-ols have been found, like catechin, epicatechin, and epicatechin gallate. Quercetin and quercetin glucoside have been identified in lesser amounts [Ballesteros-Vivas et al., 2019a; Gómez-Maldonado et al., 2020]. On the other hand, Alañón et al. [2021b] found high contents of maclurin C-glucoside, digalloylmaclurin glucoside, mangiferin, 5-galloylquinic acid, and trigalloyl glucose in the Keitt cultivar seed, and mainly hexa- and hepta-gallotannins in the Osteen variety. In the same research, iriflophenone glucoside was identified, being a characteristic benzophenone from the kernel, since it has not been found either in peel or pulp [Alañón et al., 2021b; Gómez-Caravaca et al., 2016; López-Cobo et al., 2017]. Detection of xanthones in the seed kernel is strongly linked to the presence of mangiferin being the major compound [Ballesteros-Vivas et al., 2019a; Lim et al., 2019; Torres-León et al., 2021]. Nonetheless, xanthones with other structures beside mangiferin which is C-glycoside, have been identified including mangiferin gallate and 3-O-methyl mangiferin (homomangiferin) [Ballesteros-Vivas et al., 2019a].
Roles of phenolic compounds from mango wastes in human health promotion and disease prevention
Phenolic compounds exhibit a wide range of bioactivities exerting beneficial effects on health, such as anti-allergenic, hypolipidemic, antidiabetic, antiatherogenic, anti-inflammatory, anti-microbial, antiviral, immunomodulatory, antiproliferative, anticarcinogenic, antioxidant, anti-thrombotic, cardioprotective, and vasodilatory effects, as shown in Table 2. In this context, some mechanisms of their action in preventing or reducing the risk of various chronic diseases have been discovered [Abbasi et al., 2017; Alañón et al., 2021a, b; Kim et al., 2018; Lin et al., 2020].
Table 2
Biactivities of mango by-product extracts.
Bioactivity | Extract | Mango structure | Model | Assay | Results | Reference |
---|---|---|---|---|---|---|
Antidiabetic activity | Methanol extract | Peel | In vivo | Male Wistar rats | Mango-supplemented diet exerted a significant antioxidant effect in the liver of diabetic rats, likely due to its phenolic compounds, like mangiferin. | Fernández-Ochoa et al. [2020] |
Aqueous extract | Seed kernel | In vivo | Pancreatic lipase inhibition activity (Wistar strain male albino rats) | Significant reduction in body weights, fat pad weights and organ weights of treated animals was found. Elevated levels of glucose, insulin, leptin and lipid profiles were normalized upon treatment with the extract. Antioxidant status was also brought back to normal upon treatment. Pronounced anti-obesity activity was demonstrated and this effect may be due the pancreatic lipase inhibitory activity. | Kumaraswamy et al. [2020] | |
Antioxidant activity | Ethyl acetate extracts | Peel and seed kernel | In vitro | DPPH radical scavenging activity, ferric ion reducing power (FRAP), ABTS radical cation scavenging activity, and nitric oxide radical (NO) scavenging activity. | Mango peel extracts and seed kernel extracts exhibited better radical scavenging activity compared to pulp extracts. | Kuganesan et al. [2017] |
Water and ethanol extracts | Peel | In vitro | ABTS radical cation scavenging activity and DPPH radical scavenging activity. | Extracts had high total phenolic content and possessed the high DPPH radical and ABTS radical cation scavenging activities which may be attributed to the high contents of p-hydroxybenzoic acid, gallic acid, and pyrogallol. | Huang et al. [2018] | |
Antibacterial activity | Water and ethanol extracts | Peel | In vitro | Standard method of diffusion disc plates on agar. | The extracts exhibited antibacterial activities against the five bacteria tested. The Gram-negative bacteria (Escherichia coli ATCC 11775, Salmonella typhimurium ATCC 13311, and Vibrio parahaemolyticus ATCC 17802) were more sensitive than the Gram-positive ones (Staphylococcus aureus ATCC 12600 and Bacillus cereus ATCC 14579). | Huang et al. [2018] |
Aqueous extracts | Seed | In vitro | Minimum inhibitory concentration (MIC) method and ROS assay. | Gold nanoparticles with aqueous extract efficiently inhibited the growth of Escherichia coli and Staphylococcus aureus. | Vimalraj et al. [2018] | |
Antiinflammatory activity | Ethyl acetate extracts | Peel and seed kernels | In vitro | Human Red Blood Cell (HRBC) membrane stabilization assay. | The peel and seed kernel extracts showed significantly different inhibition of hemolysis, ranging from (IC50) 151 g/mL to 197 μg/mL and from 128 to 248 μg/mL, respectively. | Kuganesan et al. [2017] |
Water and ethanol extracts | Peel | In vitro | Nitrite oxide in culture media (Murine macrophage cell lines RAW 264.7). | The results indicate that compressional-puffed ethanol extracthad apparent anti-infammatory activity, and thus it may have potential as a natural and safe agent in the protection of human health by modulating the immune system. | Huang et al. [2018] | |
Antiproliferative and anticancer activity | Ethanolic extracts | Peel | In vitro | Colorimetric assay based on the metabolic use of 3-(4,5-dimethylthiazol-2-yl)-2,5-diphenyltetrazolium bromide (MTT) by viable cells (HT29, Caco-2, and HCT116 colon cancer cells). | Already after 24 h extract treatment, viability of all the analyzed colon cancer cells was reduced in a dose-dependent manner. The effect started at 180 μg/mL of extract, and at 600 μg/mL concentration the evaluated residual viability amounted to 46%, 35%, and 44% in HT29, Caco-2, and in HCT116 cells, respectively. This effect further increased by prolonging the incubation time of cells in the presence of MPE, reaching the maximum at 48 h of treatment with 360 μg/mL dose. | Lauricella et al. [2019] |
Aqueous extracts | Seed | In vivo | Chorioallantoic membrane model (CAM) angiogenesis assay. | The result suggests that the gold nanoparticles with aqueous extract inhibited the angiogenic process by down regulating Ang-1/Tie2 pathway. | Vimalraj et al. [2018] | |
Aqueous extracts | Seed | In vitro | Human gastric cancer cell. | Biocompatibility assessment indicated the non-toxic nature of gold nanoparticles with extract towards mesenchymal stem cells at 25 μg/mL and suppressed the growth of human gastric cancer cells. | Vimalraj et al. [2018] |
Antidiabetic effects
Diabetes mellitus is one of the major metabolic diseases characterized by hyperglycemia resulting from insufficient insulin production or diminished tissue responses to insulin. In addition, cardiovascular diseases, renal failure, blindness, neurological problems, colon cancer, and others have been linked with diabetes. Hence, research about the suppression of diabetic complications is very significant. In this sense, several efforts have been made in scientific research to elucidate possible mechanisms of action of phytochemicals, such as phenolic compounds [Azhar et al., 2019; Fernández-Ochoa et al., 2020; Rodríguez-González et al., 2017]. For instance, Rodríguez-González et al. [2017] evaluated antidiabetic properties of the mango by-product, which is peel and remnant pulp. A reduction in serum glucose in diabetic rats fed mango by-product was observed, which was not associated with either intestinal absorption of glucose or protection of the islets of Langerhans. In addition, polyphenol- and carotenoid-rich extracts caused an insulin-mimetic effect on 3T3-L1 adipocyte cells and reduced the concentration of triglycerides in the serum. Likewise, diabetic nephropathy was improved by the antioxidant activity at the renal level. This antidiabetic effect was associated with the high soluble fiber content and the presence of phenolic compounds, such as ellagic acid, gallic acid, quercetin, and epicatechin gallate. In another study, consumption of mango pulp for six weeks had a beneficial effect on lean and obese individuals [Fang et al., 2018]. The effects were linked to lowering blood pressure in lean individuals and reducing levels of proinflammatory cytokines (IL-8 and MCP-1) and cardiometabolic risk biomarkers (PAI-1 and HbA1c) in obese individuals. Thereby, mango consumption reduces the risk of developing chronic obesity-related diseases, mainly by metabolite bioactivities, such as gallotannin derivatives that modulate inflammation and metabolic functions. Other phytochemicals from mango fruits and their by-products have also been linked with antidiabetic effects. In particular, mangiferin has been reported to promote glucose uptake at the cellular level through glucose transporter 4 (GLUT4) expression. It has also been linked to glycolipid metabolism enhancement, gluconeogenesis inhibition in the liver, and reduction of glucose production, blood glucose levels, and liver injury [Azhar et al., 2019; Fernández-Ochoa et al., 2020; Lin et al., 2020; Rodríguez-González et al., 2017; Soria-Lara et al., 2020]. Nonetheless, mangiferin derivatives or isomers could exhibit different mechanisms of action to promote health effects. For example, Lin et al. [2020] evaluated the effects of oral administration of mangiferin and its derivative called mangiferin calcium salt (MCS). Here, MCS exhibited better efficiency compared to mangiferin not only in the absorption process but also in the therapeutic effect in rats with type 2 diabetes and NAFLD by regulating glucose and lipid metabolism.
Mango seed kernels have also been reported to exert antidiabetic effects (in addition to their anti-tyrosinase, anti-inflammatory, anti-obesity, and hepatoprotective activities) [Abdel-Aty et al., 2018]. For example, a study by Azhar et al. [2019] disclosed a hypoglycemic effect in diabetic rats administrated 200 mg/kg of the ethanolic extract of the mango seed. There was an increase in insulin production in the pancreas and a decrease in the level of glycosylated hemoglobin (HbA1c). These effects were attributed to flavonoids and phenolic acids contained in the mango seed.
Antimicrobial effects
The phenolic compounds of mango seed have shown antifungal and antibacterial effects against Gram-positive bacteria [Asif et al., 2016; Gómez-Maldonado et al., 2020; Mutua et al., 2017]. In addition, some studies have demonstrated antimicrobial activity of gallotannins and their metabolites. For instance, gallic acid inhibited the growth of such pathogens as Clostridium perfringens that induces diseases, like e.g. colitis [Hernández-Maldonado et al., 2019; Kim et al., 2018]. In this setting, Manila mango kernel extract with phenolics has shown antimicrobial activity against Colletotrichum brevisporum [Gómez-Maldonado et al., 2020]. This in vitro study revealed that the extract (3 g/L) caused 100% inhibition of mycelial growth after 9 days and 0% spore germination after 20 h. Thereby, this evidence exposes the potential application of the mango kernel extract with phenolics as an antimicrobial agent [Gómez-Maldonado et al., 2020].
Antioxidant effects
Mangoes are endowed with considerable antioxidant properties because of their phenolic compounds, such as gallic acid, galloyl derivatives, flavonol glycosides, and benzophenones [Fang et al., 2018; Kim et al., 2017]. Notably, antioxidant activity of mango phytochemicals has been implicated in treating chronic diseases, such as diabetes, hypertension, and dyslipidemia [Mutua et al., 2017; Torres-León et al., 2021]. However, both phenolic content and antioxidant capacity in mango seem to differ across plant cultivars, fruit maturity stages, and fruit parts [Hoyos-Arbeláez et al., 2018]. Interestingly, higher antioxidant capacity has been reported in the mango peel than in the peels of other fruits [Sáyago-Ayerdi et al., 2019]. Nonetheless, the mango kernel has the highest antioxidant activity owing to gallic acid, a compound with high radical scavenging activity [Hoyos-Arbeláez et al., 2018] that was found in higher content in kernel residues from the seed compared to the peel [Alañón et al., 2021a, b; Ballesteros-Vivas et al., 2019a; Torres-León et al., 2021].
Several studies have evaluated the antioxidant activity of different fruit constituents, finding exciting results. For example, Abassi et al. [2017] evaluated cellular antioxidant activity (CAA) of various mango cultivars. They found a higher activity of peel residues than mango pulp, which contained a large content of flavonoids. Another cultivar, Xiao Tainong, with high CAA (2,986 μmol quercetin equivalent (QE)/100 g FW), has been found with compounds such as fisteine and mangiferin. Velderrain-Rodríguez et al. [2018] evaluated the antioxidant activity of three fractions of a phenolic extract from mango peel (free, acid, and alkaline fraction). The alkaline and acid fractions presented higher antioxidant capacity (DPPH/FRAP/ORAC assays) mainly attributed to the presence of free gallic acid. Recent studies have revealed that seed extracts of a “Haden” mango obtained by ultrasound-assisted extraction had a remarkable antioxidant capacity measured by DPPH, ABTS, and FRAP assays, and it was higer than that of peels. It has also been observed that changes in such variables as solvent concentration and solvent:solid ratio impact the phenolic content and affected the antioxidant capacity evaluated by different methods [Castañeda-Valbuena et al., 2021]. El-Kady et al. [2017] analyzed antioxidant activity of ethanolic extracts of seed kernel in which 20 phenolic compounds were identified and found a higher DPPH radical scavenging activity (96.86%) compared to synthetic antioxidants like – butylated hydroxytoluene (BHT) (94.9%) and ascorbic acid (91.5%). Moreover, Castro-Vargas et al. [2019] reported a 57% reduction in lipid oxidation and a 75% growth of human cancer cell lines by the antioxidant effects exerted by the phenolic extracts of mango residues, such as seed kernel. The antioxidant effect of mangiferin and other phenolic compounds from mango seed kernels has also been reported [Ballesteros-Vivas et al., 2019a].
Immunomodulation: anti-inflammatory effects
The phenolic compounds of fruits and vegetables are considered natural anti-inflammatory substances that inhibit the activation of nuclear factor κB (NF-κB). For example, anti-inflammatory activity of mango phenolics has been reported which consisted in inhibiting inducible nitric oxide synthase (iNOS), cyclooxygenase-2 (COX-2), and the tumor necrosis factor-α (TNF-α) in vitro and in vivo [Kim et al., 2017]. In this study, a significantly reduced simple clinical colitis activity index (SCCAI) was observed upon a daily intake (200-400 g) of mango pulp for eight weeks. In addition, reduced plasma levels of pro-inflammatory cytokines were detected, such as interleukin-8 (IL-8) (16.2%), growth-regulated oncogene (GRO) (25%), and granulocyte-macrophage colony-stimulating factor (GM-CSF) (28.6%) [Kim et al., 2020].
High methyl gallate content has been found in the kernel of the mango seed [Alañón et al., 2021b; Torres-León et al., 2021]. Previous studies have evidenced the anti-inflammatory activity of methyl gallate on inflammatory bowel disease [Anzoise et al., 2018]. For example, Anzoise et al. [2018] reported that methyl gallate reduced intestinal motility, decreasing peristalsis by 74.5% (dose 100 mg/kg) and 58.82% (dose 300 mg/kg), with a greater effect observed for the 100 mg/kg dose applied in a colitis model to observe the effect on inflammatory bowel disease. Likewise, a decrease in inflammatory mediators (COX-2), cytokines such as interleukin-6 (IL-6), TNF-α, and the severity of microscopic tissue damage induced by acetic acid was detected. In this same sense, pyrogallol, a metabolite produced by the microbial decarboxylation of the gallic acid present in mango, has been reported to exert anti-inflammatory activities in breast cancer and obese patients [Fang et al., 2018; Kim et al., 2018]. Likewise, the anti-inflammatory effect of mango phenolic compounds has been reported in ulcerative colitis in vitro model [Kim et al., 2017].
It is well known that phenolic compounds contribute to the modulation of the immune response by reducing inflammatory bowel disorders [Anzoise et al., 2018]. Some phytochemicals from mango residues have been linked to immunomodulatory activities. For instance, it has been noted that the consumption of mango juice by-products in children decreased the incidence of gastrointestinal and respiratory diseases, remarking the immunomodulatory properties by the modulation of immune-related proteins [Anaya-Loyola et al., 2020]. In this context, mangiferin from mango agro-industrial wastes has also been demonstrated to possess immunomodulatory properties [Alañón et al., 2021b; Lin et al., 2020; Vazquez-Olivo et al., 2019].
Antiproliferative and anticancer effects
Phenolic compounds have demonstrated antiproliferative and chemopreventive effects through modulating the cell cycle, metastasis, angiogenesis, and apoptosis. In addition, they have also been shown that significantly inhibit colon carcinogenesis by reducing oxidative stress and activating Akt and NF-κB signaling intermediates [Ballesteros-Vivas et al., 2019a; Castro-Vargas et al., 2019; Kim et al., 2017, 2018]. Several phenolic compounds of the mango peel and kernel belonging to phenolic acids, flavonoids, xanthones, benzophenones, gallotannins, and gallate derivatives have been linked to cancer prevention by antiproliferative effects on cell lines, such as leukemia, breast, lung, liver, cervix, prostate, and colon [Ballesteros-Vivas et al., 2019a; Velderrain-Rodríguez et al., 2018]. However, the biological activity of phenolics depends on their molecular structure. For example, the anticarcinogenic activity of gallotannins has been associated with the number of galloyl groups in the structure [Navarro et al., 2019]. This activity could be associated with cytotoxicity mechanisms involving apoptosis induction through an increase in Bax/ Bcl-2 protein levels, the arrest of the cell cycle in the S phase, and the inhibition of NF-κB activation, with the consequent downregulation of inflammatory cytokines [Navarro et al., 2019].
The inhibition of metastasis and proliferation of hepatocellular carcinoma (HepG2) have been evaluated using mango peel residues, observing high EC50 values of 2.35 mg/mL [Abbasi et al., 2017]. Velderrain-Rodríguez et al. [2018] also assessed the antiproliferative and the pro-apoptotic effect of mango peel extracts (free, acid, and alkaline fractions) against human colon adenocarcinoma cells (LS180) and mouse connective cells (L929). The three fractions were moderately effective against LS180 cells (IC50=~137 μg/mL), primarily to the cytotoxic effect of quercetin. Both free and alkaline fractions inhibited the growth of normal L929 cells (IC50=~197 and ~94 μg/mL, respectively). Gallic acid was the main antioxidant and responsible for the antiproliferative effect, followed by mangiferin, quercetin, and syringic acid. On the other hand, Sánchez-Camargo et al. [2021] evaluated the antiproliferative effect of the extract from mango peel containing gallic acid and some derivatives, such as galloyl esters, as well as mangiferin, gallate derivatives, quercetin, and quercetin glycosides. The results showed a high antioxidant capacity and a relevant antiproliferative activity against the human colon adenocarcinoma cell line (HT-29) after 24 h of treatment (IC50=22.98 μg/mL). In turn, Kim et al. [2018] have noted that gallic acid and pyrogallol from mango reduced cell proliferation by 37 and 78% in an in vitro model with HT-29 colon cancer cells. Also ellagic acid and ethyl gallate have been identified, which have been shown to exhibit anticancer capacity on cancer cell lines, as HTC116 and Caco-2, by the induction of apoptosis [Ballesteros-Vivas et al., 2019a].
The development of different techniques for the extraction, fractionation, and purification of phenolic compounds from the mango seed kernel has made it possible to evaluate their antiproliferative activity against the human tumor cell lines. For instance, Ballesteros-Vivas et al. [2019b] noted a significant potentiating effect on the inhibition of cell proliferation (70.51%) in the colon cell line HT-29 using an enriched phenolic extract obtained by the supercritical antisolvent fractionation (SAF) approach. Another study carried out by Castro-Vargas et al. [2019] evaluated the antiproliferative effect of a methanolic extract obtained by the traditional method from mango kernel in human cancer cell lines, such as MDA-MB-231 (breast), PC-3 (prostate), A-549 (lung), and HT-29 (colon). Surprisingly, a 75% decrease in the viability of HT-29, MDA-MB-231, and PC-3 cells was also observed.
POTENTIAL PREBIOTIC EFFECT AND ITS RELATIONSHIP WITH GUT HEALTH
Few investigations have highlighted mango residues as a potential novel source of prebiotics. Herein, we discuss the beneficial effects of phenolic compounds from mango residues on modulating the gut microbiota [Anaya-Loyola et al., 2020; Anzoise et al., 2018; Hernández-Maldonado et al., 2019; Kim et al., 2018]. Several studies have shown that the consumption of mango and by-products helps prevent gastrointestinal diseases and other related. In this context, mechanisms of action of the phenolic compounds associated with modulation of inflammation and prevention of colon cancer correlate with the gut microbiota’s functional composition [Anzoise et al., 2018; Hernández-Maldonado et al., 2019; Kim et al., 2018]. Therefore, dietary fiber and phenolics of mango residues can be considered prebiotics since both modulate the gut microbiome. In addition, the International Scientific Association of Probiotics and Prebiotics (ISAPP) recently published that phenolic compounds from plants can meet prebiotic criteria. In this sense, the ISAPP defines a prebiotic as a substrate selectively used by the host microorganism, conferring a health benefit [Anaya-Loyola et al., 2020; Hu et al., 2018; Sáyago-Ayerdi et al., 2019]. Currently, there is a plentitude of supporting evidence showing that mango and by-products consumption helps prevent gastrointestinal diseases. In this sense, it has been suggested that phytochemicals could play an essential role in the gut microbiota’s modulation by both antimicrobial and prebiotic properties.
Bioaccessibility and bioavailability of phenolic compounds
In the case of phenolic compounds, bioaccessibility refers to the phenolic fraction released from the food matrix during gastrointestinal phase digestion and made available for intestinal absorption. In contrast, bioavailability relates to the phenolic fraction or its derivatives that reach the bloodstream by intestinal absorption and become available for body functions at the site of action [Blancas-Benitez et al., 2015; Hernández-Maldonado et al., 2019; Jamar et al., 2017; Peanparkdee & Iwamoto, 2022]. Therefore, the biological contribution of phenolic compounds in the human body depends on numerous factors, such as the host condition, its gut microbiota, and the chemical and structural nature of the molecules. Firstly, the bioaccessibility and bioavailability of different phenolics vary depending on the individual’s physical condition, including digestive/absorptive/metabolic/response capability and effective dose. After ingestion, the release of phenolic compounds begins with the cellular compartmentalization and enzymatic hydrolysis of salivary α-amylases. Then, in the gastric phase, they interact with endogenous enzymes influencing the digestibility of the components of the food matrix, such as starch (α-amylase), lipids (pancreatic lipase), and proteins (pepsin and trypsin) [Peanparkdee & Iwamoto, 2022; Wu et al., 2017]. Then, multiple enzymes endowed with diverse metabolic capacities in the human gut microbiome contribute to the absorption of phenolics by their transformation or degradation [Bento-Silva et al., 2020; Blancas-Benitez et al., 2015; Hernández-Maldonado et al., 2019; Jamar et al., 2017; Pacheco-Ordaz et al., 2018a; Vazquez-Olivo et al., 2019; Velderrain-Rodríguez et al., 2018]. However, it has been reported that up to 65% of anthocyanins present in the diet are not absorbed in the gastrointestinal tract [Tian et al., 2019]. The phenolic compounds’ absorption also depends on their molecular structure, composition (free form or interacting with another component), and the degree of polymerization, which influences their solubility, permeability, and molecular stability.
Many authors have focused on knowing the bioaccessibility and bioavailability of mango phenolic. For instance, Blancas-Benitez et al. [2015] determined the phenolic bioaccessibility in the mango peel using an in vitro digestion system. As a result, a relative bioaccessibility of 43.53% was found. The vanillic acid and hydroxycinnamic acids were accessible for absorption in the small intestine and available to exert beneficial health effects. Hernández-Maldonado et al. [2019] found that 54% of the phenolic compounds in a mango-based bar were absorbed during the gastrointestinal phase, while 46% were hydrolyzed in the colon. Both research results regarding low bioaccessibility could be associated with the instability of the compounds that tend to degrade during intestinal digestion, such as phenolic acids, catechin, quercetin, resveratrol, and rutin [Peanparkdee & Iwamoto, 2022; Tian et al., 2019]. In this same sense, resistant compounds that reach the small intestine have also been observed. For example, Velderrain-Rodríguez et al. [2018] determined the absorption probability of phenolics from the Ataulfo mango peel in enterocytesin. They found a wide range of compounds with different relative absorption rates, such as p-coumaric and 2-hydroxycinnamic acid (89%), ferulic acid (86%), syringic acid (83%), protocatechuic acid (82%), gallic acid (75%), catechin (71%), quercetin (64%), ellagic acid (60%), mangiferin (40%), and rutin (16%). Herein, gallic acid and derivatives showed an absorption percentage higher than 57%, indicating better cell permeability. This result could potentially be associated with the antiproliferative effect reported for gallic acid. In addition, Pacheco-Ordaz et al. [2018a] evaluated the acid and alkaline hydrolysis of Ataulfo mango peel extracts using Caco-2 cell monolayers as a model for intestinal epithelial permeability. They reported gallic acid from an alkaline fraction presented an apparent permeability coefficient of 2.61×10-6 cm/s compared to the standard of 2.48×10-6 cm/s. Overall, the results suggest that most phenolic compounds (except for rutin) could be considered bioaccessible at the intestinal level; and some compounds could be even absorbed.
The conjugation of hydroxybenzoic acids limits bioaccessibility and bioavailability of some oligomers, such as gallotannins and ellagotannins formed from gallic acid and ellagic acid, respectively [Bento-Silva et al., 2020; Velderrain-Rodríguez et al., 2018]. Under gastrointestinal phase conditions, these compounds are hydrolyzed to simple molecules, like gallic acid [Anaya-Loyola et al., 2020]. However, branched-chain tannins that are not absorbable in their native form can be hydrolyzed and decarboxylated by microbial enzymes. The resulting metabolites, such as gallic acid and pyrogallol, can be absorbed in the colon [Kim et al., 2018]. In this same sense, mangiferin also is a compound with low absorption and little bioavailability compared to other phenolic components. Thereby, it should form complexes with other molecules to improve its solubility, food release, bioavailability, and other properties [Lin et al., 2020; Velderrain-Rodríguez et al., 2018]. However, compounds such as mangiferin gallate can undergo hydrolysis during gastric digestion, which leads to the mangiferin release [Hernández-Maldonado et al., 2019]. On the contrary, hydroxycinnamic acids and kaempferol require intestinal enzymes for absorption. In this regard, chlorogenic acid is a compound with low intestinal absorption. However, its bioavailability depends also on the metabolism of the gut microbiota. It has even been reported that it promotes the modulation of the colonic microbiota through stimulating the growth of Bifidobacterium spp., Clostridium coccoides, and Eubacterium rectale [Coman & Vodnar, 2020]. Therefore, these scientific works suggest that the mango wastes’ phenolic compounds can be modified not only to enhance their intestinal absorption but also to achieve their profiles enhancing the gut microbiota.
Understanding the bioaccessibility and bioavailability of phenolic compounds is crucial to establishing a functional characterization. For that reason, many researchers have focused on deciphering these mechanisms through different approaches. For instance, a significant effect on the release of phenolic compounds during an in vitro gastrointestinal simulation was observed in a study using a mango product with high total soluble phenolic contents. Herein, gastric digestion showed a release rate of 9% higher than the intestinal phase [Hernández-Maldonado et al., 2019]. These results could be associated with covalent interactions of the phenolic compounds with the polysaccharides of the cell wall [Hernández-Maldonado et al., 2019; Wu et al., 2017]. In addition, glycosidic bonds from polyphenols can be broken down by acid hydrolysis, causing the release of aglycones [Pacheco-Ordaz et al., 2018a].
In the intestinal section, the release of phenolic compounds is affected by pH changes and enzymes activity (mainly pancreatin and α-amylase). Both factors weaken molecular interactions in the food matrix, promoting bond hydrolysis. Particularly, intestinal esterases hydrolyze ester bonds between carbohydrates linked to phenolic compounds and other cell wall components in plants, increasing the bioavailability of phenolic compounds. They may be then absorbed by the gastrointestinal barrier and get into blood circulation. Generally, phenolic compounds are not fully absorbed by their structural complexity, and around 90% of these compounds reach the colon where they are fermented by intestinal microorganisms. Here, about 2,671 microbial species exist, constituting the human gut microbiota [Lagier et al., 2018; Ye et al., 2022]. These microorganisms are genetically endowed with enzymes such as esterases, lipases, carbohydrates, and proteases. Among them, α-l-rhamnosidases, β-d-glucosidase, and β-d-glucuronidases allow the release, absorption, and production of metabolites, such as bioactive phenolic acids, including gallic acid, protocatechuic acid, syringic acid, and vanillic acid [Bento-Silva et al., 2020; Coman & Vodnar, 2020; Hernández-Maldonado et al., 2019; Jamar et al., 2017; Pacheco-Ordaz et al., 2018a; Tian et al., 2019].
Interestingly, a recent study evaluated the phenolic compounds’ bioaccessibility in a mango pulp and peel-based bar during gastrointestinal phase and after fermentation [Hernández-Maldonado et al., 2019]. The results of this research identified not only phenolic acids (gallic, ferulic, coumaric, and caffeic acid) but also flavonoids and xanthones (quercetin, gallocatechin, mangiferin, and mangiferin gallate), reporting their 53.78% bioaccessibility. Likewise, products after fermentation were hydroxyphenolic acids and acetic acids as the primary shortchain fatty acids. In addition, norathyriol classified as xanthone (aglycone) was identified after 12 h of fermentation. Mango peel’s total soluble phenolic bioaccessibility has been reported at 44%. Soluble and released by hydrolysis phenolics represent only 11.46% of the insoluble fiber content. Hence then, nonbioaccessible and released soluble phenolics can reach the colon and potentially be used as substrates by the colonic microbiota [Hernández-Maldonado et al., 2019; Sáyago-Ayerdi et al., 2019]. Thereby, the insoluble fiber composition can influence microbiota composition changes during the fermentation process.
The gut microbiota plays a critical function in the biotransformation of phytochemicals, modulating the phenolic compounds’ bioavailability and promoting gut health [Bento-Silva et al., 2020; Hernández-Maldonado et al., 2019; Kim et al., 2018]. Therefore, the consumption of probiotics, prebiotics (dietary fiber), and phenolic compounds is recommended for better gut health, considering that phenolic molecules have the same biological effect as prebiotics [Hernández-Maldonado et al., 2019; Ye et al., 2022].
Gut health and modulation of the gut microbiota
The intake of fruits and vegetables is closely related with gut health because their bioactive compounds exert beneficial effects, such as phenolic compounds that remain bioaccessible after the gastrointestinal phase in the non-digestible fraction [Hernández-Maldonado et al., 2019; Kim et al., 2020; Pacheco-Ordaz et al., 2018a]. Hydroxycinnamic acids, gallotannins, and other compounds interacting with the fiber have been found therein [Hernández-Maldonado et al., 2019; Sáyago-Ayerdi et al., 2019; Velderrain-Rodríguez et al., 2018]. Also flavonoids and xanthones associated with the insoluble fiber fraction have been identified in mango, including quercetin, kaempferol, and mangiferin. Notably, as shown by assays with the fecal microbiota, this flavonoid-xanthone fraction produces compounds that benefit gut health, such as hydroxyphenolic acids and other derivatives [Hernández-Maldonado et al., 2019; Kim et al., 2018].
Regarding gastrointestinal infectious diseases, dietary fiber intake has been shown to help mitigate disorders, including constipation and diarrhea associated with gut microbiota changes. In addition, dietary fiber is related to bioactive components such as phenolic compounds, which are bioaccessible once released in the digestive tract [Anaya-Loyola et al., 2020; Hernández-Maldonado et al., 2019]. Thereby, diarrhea reduction can be associated with the content of gallotannins, such as penta-, hexa- and heptagalloyl glucose, in mango residues, which inhibit the growth of pathogenic microorganisms, like Campylobacter jejuni involved in acute diarrhea [Anaya-Loyola et al., 2020; Kim et al., 2018; Vazquez-Olivo et al., 2019].
In another study, a reduction in flatulence and abdominal inflammation was reported in children who consumed a beverage from mango juice residues (peel and pulp), which was constituted by dietary fiber with a high content of extractable polyphenols (mono- to heptagalloyl hexosides and mangiferin) and polyphenols released by hydrolysis [Anaya-Loyola et al., 2020]. Methyl gallate has also been demonstrated to exert antidiarrheal, spasmolytic, anti-inflammatory, and antioxidant effects in preclinical models of intestinal inflammation [Anzoise et al., 2018]. The cited authors also showed it reduced intestinal motility, decreasing peristalsis by 74.5% (100 mg/kg) and 58.82% (300 mg/kg), and observed a greater effect of the 100 mg/kg dose applied in a preclinical model with intestinal disorders.
Multiple factors are involved in the gut health, such as a balanced microbial composition, intestinal permeability, healthy epithelial barrier, and a vigorous colonic mucosa. Any disorder of these factors can cause oxidative stress and induce development of intestinal diseases, including obesity, inflammatory bowel disease, mucosal ulceration, ulcerative colitis, and Crohn’s disease [Hernández-Maldonado et al., 2019; Kim et al., 2020; Ye et al., 2022]. Particularly, inflammatory bowel disease is characterized by chronic inflammation and dysbiosis, which are the main factors for the development of gastrointestinal neoplasms or colon cancer. Worthy of mention is that 60% of stomach cancer and 43% of colon cancer are attributed to low consumption of vegetables [Anzoise et al., 2018; Kim et al., 2018; Navarro et al., 2019; Vazquez-Olivo et al., 2019].
The gut microbiota plays an important role in food digestion, immunity and other metabolic functions. The human intestinal microbial communities are defined by host genetics, intestinal dysbiosis, endogenous factors (antibiotics and xenobiotics consumption), and other environmental factors. However, dietary components play a crucial role in regulating microbial profiles, mainly fiber and phenolic compounds [Bento-Silva et al., 2020; Kim et al., 2018]. From this perspective, phenolic compounds have become relevant for health since they can modify the gut microbiota, increasing the release of metabolites with more significant bioactivity [Hernández-Maldonado et al., 2019; Kim et al., 2018]. Moreover, phenolic molecules with a higher degree of polymerization, as gallotannins, have been linked with substantial anti-inflammatory and anticancer properties [Navarro et al., 2019]. For example, Kim et al. [2020] demonstrated that gallotannins and gallic acid mitigated intestinal inflammation and carcinogenesis by intestinal microbiome modulation. Mangiferin has also been reported to improve intestinal inflammation in a murine model with induced colitis [Kim et al., 2018; Vazquez-Olivo et al., 2019]. On the other hand, Navarro et al. [2019] identified 71 compounds, including 32 gallates and gallotannins with different degrees of polymerization, 7 hydroxybenzophenone derivatives (maclurin and iriflophenone), 6 xanthonoids (isomangiferin derivatives and mangiferin), 11 phenolic acids, and 8 flavonoids (derived from rhamnetin and quercetin), when evaluating the cytotoxic effect of mango peel extracts on different human carcinoma cells related to the digestive tract, and concluded that gallotannins and xantonoids play an essential role in toxicity against cancer cells.
The mango’s phenolic compounds and their derivatives have also been noted to participate in processes linked to immune system regulation by gut microbiota modulation. For instance, using a rat model with DSS-induced colitis, Kim et al. [2020] surveyed a mango polyphenol concentrate. They found significant changes in the profiles of bacteria belonging to the Firmicutes Phylum (Lactobacillus plantarum, Lactococcus lactis, and Clostridium butyrium). Consequently, the production of short-chain fatty acids (SCFAs) was induced as butyrate and valerate. It was also observed a suppression of HDAC1 activity and an increase of AMPK activity together with levels of autophagy biomarkers; thereby, the mango phenolic compounds intake could mitigate intestinal inflammation. In addition, other studies have demonstrated that quercetin can modify the gut microbiota, improving the Bifidobacteria growth and the ratio of Firmicutes decreasing to Bacteroidetes. Interestingly, by conducting an in vitro gut simulation to evaluate a mango by-product rich in polyphenols and dietary fiber, Sayago-Ayerdi et al. [2019] detected an increase in Bifidobacterium relative abundance at 24 h and increased microbial genera diversity at 72 h, including mainly Lactobacillus, Dorea, and Lactococcus. In this sense, through a human clinical study, Kim et al. [2018] showed that mango intake encouraged the growth of Lactobacillus genera (L. plantarum, L. reuteri, and L. lactis), promoting butyrate production. Furthermore, mango consumption mitigated intestinal inflammation by decreasing the production of pro-inflammatory cytokines.
APPLICATIONS OF AGRO-INDUSTRIAL WASTES
New research challenges have opened an approach to food applications of valuable waste products. In this context, functional foods or dietary supplements have been designed with mango by-products implemented as active ingredients [Alañón et al., 2021a; Anaya-Loyola et al., 2020; Ballesteros-Vivas et al., 2019a; Oliver-Simancas et al., 2021]. Currently, the trend of developing new products in various sectors of the food industry, such as chicken patties and meat sausages in the meat industry, as well as dairy-based drinks and cheeses in the dairy industry, that are based among others on industrial by-products has been increasing [Gupta et al., 2022; Iuit-González et al., 2019; Oliver-Simancas et al., 2021]. Several products have been reported to be developed from mango wastes, such as macaroni, noodles, films, yogurt, beverages, and bakery food products, like snacks, bread, biscuits, cakes, and mango-based bars [Abbasi et al., 2017; Hernández-Maldonado et al., 2019; Marcillo-Parra et al., 2021; Melo et al., 2019]. Mango seed kernel has been deployed to develop biodegradable films, edible coatings for tomato [Nawab et al., 2017] and peach [Torres-León et al., 2018], as packaging in chili [Nawab et al., 2018], and to prepare extruded and deep-fried pellets [Patiño-Rodríguez et al., 2021]. It has been reported that phenolic compounds present in mango by-products preoved well as probiotic enhancers that can be applied in yogurts, cheeses, olives, sauerkraut and other functional foods [Pacheco-Ordaz et al., 2018b; Tirado-Kulieva et al., 2021]. Pérez-Chabela et al. [2022] evaluated the effect of mango peel as a functional ingredient in yogurt. They observed that physicochemical characteristics, such as titratable acidity and pH, were accepted by consumers. The addition of this mango by-product had an effect because it inhibited undesirable microorganisms and promoted the proliferation of lactic acid bacteria, indicating that it presents prebiotic capacity. On the other hand, Das et al. [2019] incorporated up to 40% of mango kernel flour in the formulation of cakes and compared them with those made with 100% wheat flour. They observed that mango kernel flour affected the nutritional composition of the cakes. In addition, Iuit-González et al. [2019] developed a jam based on mango peel, evaluating the incorporation of 20 and 30%, the latter being the most preferred by the panelists. They indicated that the mango jam complied with the specifications of the Mexican Official Standard No. NOM-130-SSA1-1995 [Norma Oficial Mexicana, 1997]. Mango by-products have also been used as vegetable oil stabilizers to prevent oxidation [El-Kady et al., 2017, Sánchez-Camargo et al., 2019]. Thereby the synthetic antioxidants linked to harmful health events, such as butylhydroxyl anisole and butylated hydroxytoluene, could be substituted with antioxidants from natural sources. Another significant finding on mango residues is their antimicrobial properties, which allow their application as antimicrobial agents to prevent food poisoning and infections caused by pathogenic microorganisms in the food industry [Mutua et al., 2017].
Understanding the underlying mechanisms of bioactive components from mango wastes opens new pharmacotherapy treatment options to avoid side effects associated with chronic-degenerative diseases [Marcillo-Parra et al., 2021]. On the other hand, phenolic extracts from mango residues have also been used in combined therapy with conventional drugs to treat gastrointestinal diseases due to their potential to reduce levels of inflammatory biomarkers and modulate the gut microbiota [Hernández-Maldonado et al., 2019; Kim et al., 2020].
Overall, mango residues are a low-cost source of phenolic compounds applicable in the food, cosmetic, and pharmaceutical industries, allowing the increase in the valorization of these residues and the reduction of their environmental effects [Alañón et al., 2021a; Borrás-Enríquez et al., 2021; Castro-Vargas et al., 2019; El-Kady et al., 2017; Lin et al., 2020; Marcillo-Parra et al., 2021; Mutua et al., 2017; Torres-León et al., 2021].
CONCLUSION
Mango processing industries bring about a massive volume of residues causing environmental problems. Nonetheless, several phytochemicals in the peel and seed kernel of the mango have been linked to preventing several diseases, such as cancer, cardio-metabolic diseases, and gastrointestinal diseases. The molecules present in mango waste have been reported to be involved in the gut microbiota modulation showing a prebiotic effect and improving gut health. These therapeutic effects make the mango by-products viable bioactive ingredients for use in the design of functional and nutraceutical foods for health improvement.