INTRODUCTION
Phenolics have been shown to potentially elicit beneficial effects on human health [Hung et al., 2015; Khan et al., 2019], but they need to be released from the food matrix in the digestive tract and then absorbed to show those effects. The quantity of phenolics released in the digestive tract which become available for absorption is called bioaccessibility [Palafox-Carlos et al., 2011]. Since bioaccessibility is important in the explanation of the beneficial effects of phenolics, it has been studied in recent years [Lingua et al., 2019; Quatrin et al., 2020; Solari-Godiño et al., 2017; Stübler et al., 2020]. The conditions in the digestive tract, such as pH, and the presence of various food components can affect the phenolics bioaccessibility [Palafox-Carlos et al., 2011]. Studies have shown that proteins, lipids or carbohydrates affect phenolics release in the digestive tract [Jakobek, 2015] due to their interactions with phenolics.
Dietary fiber intake is commonly part of a human diet. Plant fibers are resistant to digestion in the small intestine but can be digested in the lower parts of the digestive tract by the activity of microflora [Saura-Calixto, 2011] and can interact with various food components. Fibers can interact with phenolics, which was shown in the case of polyphenols from blackberry purée [Tomas et al., 2020] or anthocyanins [Larsen et al., 2019]. By interacting with phenolics, fibers can “carry” them to the lower parts of the digestive tract [Saura-Calixto, 2011].
β-Glucans are soluble dietary fibers which are present in certain cereals [Mäkelä et al., 2020] which also elicit health-beneficial effects. The presence of β-glucans can cause the reduction in the rate of intestinal transit of nutrients, which can be helpful in the attenuation of blood glucose levels [Mäkelä et al., 2020]. Studies have also found that β-glucans can interact with phenolics [Gao et al., 2012; Jakobek et al., 2021]. During such interactions, phenolics might adsorb onto β-glucans [Gao et al., 2012; Jakobek et al., 2021], while β-glucans potentially provide a system that can “carry” phenolics through the digestive tract and “deposit” potentially high concentrations of phenolics to the lower parts of the digestive tract. There has been a study of the behavior of β-glucans and phenolics during in vitro digestion and colonic fermentation of barley and barley products containing both components [Mosele et al., 2018]. Knowledge of the interaction of phenolics from other sources with β-glucan in the digestion process is, however, limited.
Aronia or black chokeberry (Aronia melanocarpa) is rich in phenolics [King & Bolling, 2020; Sidor et al., 2019], which makes this fruit popular to produce various food products and dietary supplements. Since aronia is used increasingly in the human diet due to its potential beneficial effects [King & Bolling, 2020; Sidor et al., 2019], knowledge of the fate of aronia phenolics in the digestive tract and their bioaccessibility is needed. It is known that aronia phenolics can adsorb onto β-glucans [Jakobek et al., 2021]. However, there are no previous studies of the interactions of β-glucans and aronia phenolics in the digestion process.
The aim of this study was, therefore, to investigate the interactions between phenolics from aronia and β-glucans by studying the in vitro simulated gastrointestinal digestion of aronia phenolics, with and without added β-glucans, and by studying the adsorption process between aronia phenolics and β-glucans.
MATERIALS AND METHODS
Study design
The in vitro simulated digestion of aronia was conducted without β-glucan and with 15 and 30 mg/L of β-glucan added in the digestion process. In addition, the adsorption of aronia phenolics onto β-glucan was conducted with β-glucan concentrations of 15 and 30 mg/L.
Chemicals
Reagents were purchased from Gram mol, Zagreb, Croatia (KCl, KH2PO4, NaHCO3, CaCl2, MgCl2×6H2O); from Kemika, Zagreb, Croatia ((NH4)2CO3, Na2HPO4×12H2O, NaH2PO4×2H2O, CH3COONa×3H2O); from Carlo Erba Reagents, Val de Reuil, France (NaCl); from Fluka, Buchs, Switzerland (o-phosphoric acid 85% HPLC-grade); from J.T. Baker, Gliwice, Poland (methanol, CH3COOH); from Fisher Scientific, Loughborough, UK (acetonitrile); and from Avantor, Arnhem, Netherlands (HCl). α-Amylase (13 U/mg), pepsin (632 U /mg), pancreatin (8 USP), quercetin 3-rutinoside hydrate, quercetin 3-glucoside, chlorogenic acid, bile salt, and barley β-d-glucan were purchased from Sigma-Aldrich, St. Louis, MO, USA. Cyanidin 3-galactoside chloride, cyanidin 3-glucoside chloride, and quercetin 3-galactoside, were obtained from Extrasynthese, Genay, France.
Preparation of reagents
Stock solutions of electrolytes were dissolved in distilled water [Minekus et al., 2014]: KCl (0.5 M), KH2PO4 (0.5 M), NaHCO3 (1 M), MgCl2 (0.15 M), (NH4)2CO3 (0.5 M), NaCl (2 M), and CaCl2 (0.3 M). Simulated salivary fluid, simulated gastric fluid, and simulated intestinal fluid were prepared in volumetric flasks by pipetting appropriate volumes of stock solutions of electrolytes and adding water to make up the final volume of flasks. The final concentrations of electrolytes in the simulated salivary fluid were: 18.87 mM KCl, 4.62 mM KH2PO4, 17 mM NaHCO3, 0.056 mM MgCl2, and 0.06 mM (NH4)2CO3[Minekus et al., 2014]. In the simulated gastric fluids, the concentrations were: 8.62 mM KCl, 1.12 mM KH2PO4, 31.25 mM NaHCO3, 0.15 mM MgCl2, 0.62 mM (NH4)2CO3, and 59.00 mM NaCl [Minekus et al., 2014]. The simulated gastric fluid was adjusted to pH 3 with 1 M HCl. In the simulated intestinal fluid, the final electrolyte concentrations were: 8.5 mM KCl, 1 mM KH2PO4, 106.25 mM NaHCO3, 0.41 mM MgCl2, and 48.00 mM NaCl [Minekus et al., 2014]. The simulated intestinal fluid was adjusted to pH 7 with 1 M HCl. Enzyme solutions, α-amylase (1.00 g/L), pepsin (31.66 g/L), and pancreatin (8.00 g/L), were prepared in simulated salivary, gastric and intestinal fluids, respectively. β-Glucan was prepared in distilled water (0.55 g/L and 1.10 g/L) and bile salt in the simulated intestinal fluid (25.00 g/L). All solutions were prepared daily and pre-incubated at 37°C before use. Solutions of pH 1.5 (HCl/KCl), pH 3.0 (citrate buffer, CH3COONa/CH3COOH), and pH 7.0 (phosphate buffer, Na2HPO4/NaH2PO4) were prepared as 0.1 M.
Phenolic extraction
One kg of aronia (A. melanocarpa) was harvested from orchards in Croatia (Orahovica), immediately frozen and stored at –18°C. Aronia was homogenized with a stick blender (FA 5295, First, Austria).
For chemical extraction [Jakobek et al., 2021], aronia (3 g) and 22.5 mL of 80% (v/v) methanol were placed in a plastic tube, vortexed and extracted in an ultrasonic bath (Bandelin Sonorex RK 100, Berlin, Germany) for 15 min. The mixture was centrifuged (SL 8R, Thermo Fisher Scientific, Waltham, MA, USA) for 10 min at 12,108×g. The liquid part was separated from the fruit residue, and the residue was extracted again using 10 mL of 80% (v/v) methanol, following the same procedure as above (ultrasonic bath 15 min, centrifuge 10 min at 12,108×g, supernatant separation from the residue). The two supernatants were combined. An aliquot was filtered (0.22 μm polytetrafluoroethylene (PTFE) filter) and analyzed using the high-performance liquid chromatography (HPLC) method as described below.
The fruit residue that remained after the chemical extraction was extracted with an enzyme-assisted extraction to extract the remained phenolics bound to dietary fiber [Bergantin et al., 2017] as follows: 21 mL of distilled water, 1.2 mL of bile salt, 0.6 mL of pancreatin, and 0.3 mL of pepsin were added into the fruit residue. The solution was vortexed, incubated for 2 h at 37°C in a water bath while shaking (SW 22, Julabo, Seelbach, Germany), and centrifuged at 5°C at 12,108×g for 5 min. The supernatant was separated from the residue. The residue was extracted again with the same procedure. An aliquot (1 mL) of both supernatants obtained by enzymatic extractions was filtered through a 0.22 μm PTFE filter, put on ice, and analyzed separately using the HPLC method. The final total content of phenolics in aronia was calculated as a sum of contents determined after the chemically-assisted and the two enzyme-assisted extractions, and represented the content of phenolics before digestion.
Simulated digestion
For the oral phase digestion, a homogenized aronia was weighed (3 g) in a plastic tube where after 3.5 mL of simulated salivary fluid, 975 μL of H2O, 25 μL of CaCl2 (0.3 M), and 500 μL of α-amylase was pipetted. The solution was vortexed for 30 s and then put on ice. An aliquot of 500 μL was taken, filtered through a 0.22 μm PTFE filter, and analyzed using the HPLC method. To the solution remaining after the oral phase, 7.5 mL of simulated gastric fluid, 295 μL of H2O, 5 μL of CaCl2(0.3 M), 200 μL of HCl (1 M), and 2 mL of pepsin were added to simulate the digestion. The solution was vortexed, incubated in a water bath with shaking for 2 h at 37°C, centrifuged at 5°C at 12,108×g for 5 min, and put on ice. An aliquot of 500 μL was taken, filtered through a 0.22 μm PTFE filter, and analyzed using the HPLC method. Then, the intestinal phase digestion was carried out. The solution remaining after the gastric phase digestion was treated by adding 11 mL of simulated intestinal fluid, 3.61 mL of H2O, 40 μL of CaCl2(0.3 M), 150 μL of NaOH (1 M), 5 mL of pancreatin, and 0.2 mL of bile salt. The solution was vortexed, incubated for 2 h at 37°C in a water bath with shaking, centrifuged at 5°C at 12,108×g for 5 min, and put on ice. One mL was filtered through a 0.22 μm PTFE filter, and analyzed using the HPLC method. The simulated digestion of aronia with β-glucan was conducted with the same described procedure. The only difference was the addition β-glucan during all three steps of digestion so that its final concentration was 15 mg/L. A stock solution of β-glucan (0.55 g/L) was used. The digestion process was repeated without and with 30 mg/L of β-glucan with the same described procedure. The stock solution of β-glucan was 1.10 g/L.
The percentage of recovered phenolics was calculated using Equation 1:
where: γdigestion phase is the content of recovered phenolics after a particular digestion phase (mg/kg fresh weight (FW)) and γbefore digestion is the phenolics content in fruit before digestion determined after chemically- and enzyme-assisted extraction (mg/kg FW).Reversed-phase high performance liquid chromatography (RP-HPLC) analysis
Samples were analyzed on the HPLC system (1260 Infinity II, with a quaternary pump, a PDA detector and a vial sampler) (Agilent Technology, Santa Clara, CA, USA). Phenolics were separated using a Poroshell 120 EC C-18 column (4.6×100 mm, 2.7 μm) protected by a Poroshell 120 EC-C18 guard column (4.6 mm). The mobile phase consisted of 0.5% (w/v) H3PO4 (A) and 100% acetonitrile (B). Phenolics were separated with a gradient of 5–11% B 0–5 min, 11–15% B 5–7.5 min, 15–17.5% B 7.5–17.5 min, 17.5–20% B 17.5–20 min, 20–30% B 20–30 min, 30–70% B 30–32 min, 70% B 32–34 min, 70–5% B 34–36 min, and 5% B 36–38 min. The flow rate was set to 0.8 mL/min, and the volume of injection was 10 μL. The identification of phenolics was done by comparing the UV/Vis spectra and retention times of peaks with those of authentic standards. Samples were spiked with authentic standards to confirm the identification. Quantification was done by using calibration curves of authentic standards. Calibration curves were linear (r2 0.9942 to 0.9991). Some phenolics (neochlorogenic acid, cyanidin 3-arabinoside and cyanidin 3-xyloside) were tentatively identified by using literature data [Sosnowska et al., 2018], and quantified by using chlorogenic acid and cyanidin 3-glucoside calibration curves. Precision was determined by calculating the coefficient of variation of several measurements of phenolic standards (0.3 to 13.1%).
Adsorption capacity determination
The adsorption was studied at pH 1.5, 3.0, and 7.0, for 4 h (chosen due to the total duration of gastric and intestinal phase), at 37°C (the temperature of the human body and simulated digestion). The pH 3.0 and 7.0 were chosen for the adsorption study to match the pH values of simulated gastric and intestinal fluids, respectively. In addition, aronia contains high concentrations of anthocyanins whose chemical structures change depending on the pH. To cover wider pH range and different chemical structures of anthocyanins, the adsorption was also studied at pH 1.5. At such low pH, anthocyanins are considered stable and they take the form of a flavylium cation, while their structure changes at higher pH.
The reaction solution of 1.5 mL consisted of an aronia extract obtained with chemical extraction (50 μL), an aliquot of β-glucan to give a final concentration of 15 mg/L and an appropriate volume of buffer (pH 1.5, 3.0 and 7.0). Solutions were put in an incubator for 4 h at 37°C (IN 30, Memmert, Schwabach, Germany), and centrifuged at 6,739×g for 10 min (Eppendorf Minispin, Eppendorf, Hamburg, Germany). An aliquot of 1 mL was taken from the reaction solution, filtered through a 0.22 μm PTFE syringe filter, and analyzed via HPLC to determine un-adsorbed phenolics (ce, mg/L). The adsorption was repeated for β-glucan at a concentration of 30 mg/L.
The adsorption capacity (qe) (mg of phenolics adsorbed onto g of β-glucan) was calculated using Equation 2:
where: c0 is the initial phenolic concentration in the reaction solution (mg/L), ce is the concentration of un-adsorbed phenolics (mg/L), Vm is the total volume of the reaction solution (L), γa is the β-glucan concentration (g/L), and Va is the volume of added β-glucan in the reaction solution (L).Zeta potential measurement
Aronia extract obtained after chemical extraction was diluted 1:30 (v/v) in buffers of pH 1.5, 3.0 and 7.0. β-Glucan was prepared in the same buffers with a final concentration of 15 and 30 mg/L. A mixture of aronia – β-glucan was prepared by mixing the aronia extract and β-glucan in the same buffers (aronia extract diluted 1:30 (v/v), β-glucan at 15 and 30 mg/L). All solutions were put in an incubator at 37°C for 4 h. Zeta potential was measured on a Zetasizer 2000 analyzer (Malvern, Malvern, UK).
Statistical analysis
All experiments were performed in triplicate each measured once (n=3). Analysis of variance (ANOVA) with post-hoc Tukey test was used to analyze differences between the results (Minitab LLC., State College, PA, USA). Principal component analysis was used for possible clustering of the data (Minitab LLC).
RESULTS AND DISCUSSION
Simulated digestion
Flavonols (quercetin 3-rutinoside, quercetin 3-galactoside, and quercetin 3-glucoside), anthocyanins (galactoside, glucoside, arabinoside, and xyloside of cyanidin) and phenolic acids (neochlorogenic acid and chlorogenic acid) were quantified in aronia extract (Table 1). This profile of phenolics was similar to that determined in previous studies [Denev et al., 2019; Sosnowska et al., 2018].
Table 1
The percentage of recovered phenolics from aronia (before digestion) after the oral, gastric and intestinal phase of digestion without or with added β-glucan (15 and 30 mg/L).
The aronia extract was subjected to the in vitro simulated digestion process without or with added 15 mg/L and 30 mg/L of β-glucan (Table 1). Individual phenolics were recovered in all phases of digestion significantly (p<0.05) below 100% (aronia before digestion) with expiation of chlorogenic acid in the oral phase with 15 mg/L β-glucan (94.1%) and the gastric phase without β-glucan (94.7%). This is similar to the simulated digestion of apples [Bouayed et al., 2011; Fernández-Jalao et al., 2020] or grapes [Lingua et al., 2019] where the quantities of recovered phenolics were lower than the original quantities before the digestion.
Figure 1
The percentage of recovered phenolics of individual subclasses and total phenolics from aronia after the oral, gastric, and intestinal phase of digestion A) without added β-glucan (part of the experiment with data on graph B), B) with added β-glucan at concentration of 15 mg/L, C) without added β-glucan (part of the experiment with data on graph D), and D) with added β-glucan at concentration of 30 mg/L. The significant differences between recovery in the oral, gastric, and intestinal phase according to post-hoc Tukey test (p<0.05) are marked by different letters above bars. The data (mean and standard deviation) was obtained in three independent experiments, each measured once (n=3).
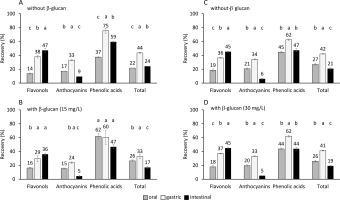
Figure 1 shows the percentage of recovery of total phenolics in each step of digestion. Total phenolics were recovered in the oral digestion step in 22 and 26% without or with added 15 mg/L of β-glucan, respectively, and in 27 and 26% without or with added 30 mg/L of β-glucan, respectively. In comparison to the oral digestion, the recovery in the gastric digestion phase increased significantly (p<0.05)) to 44 and 33% without or with added 15 mg/L of β-glucan, respectively, and to 42 and 41% without or with added 30 mg/L of β-glucan, respectively. After the gastric phase, the recovery decreased significantly (p<0.05)) in the intestinal phase to 24 and 17% without or with added 15 mg/L of β-glucan, respectively, and to 21 and 19 % without or with added 30 mg/L of β-glucan, respectively. In comparison to the total phenolic recovery in the oral phase, the total phenolic recovery in the gastric phase increased (Figure 1). This increase might be caused by the longer gastric phase, which lasted 2 h, in comparison to the oral phase, which lasted only 30 s. However, phenolics might also biodegrade/hydrolyze as they are sensitive to the alkaline environment of the small intestine, which can cause their recovery in the small intestine to decrease. The degradation of phenolics into products with different chemical structures can be suggested, as was mentioned in earlier studies [Bermúdez-Soto et al., 2007; Bouayed et al., 2011, 2012; Fernández-Jalao et al., 2020]. In addition, the percentage recoveries are similar to other studies [Bouayed et al., 2011; Lingua et al., 2019; Solari-Godiño et al., 2017]. The recoveries of total phenolics from grapes (34, 37, 13% after oral, gastric and intestinal phases, respectively) [Lingua et al., 2019] or anchovy mince with added phenolics (30 to 54% after intestinal digestion) [Solari-Godiño et al., 2017] are comparable to our study results. High percentages of phenolics have been also recovered from apples, i.e., approx. 65 and 75% after gastric and intestinal phases, respectively [Bouayed et al., 2011].
Flavonols
The recovery of total flavonols in the oral phase of digestion was 14 and 16% in the case of the digestion conducted without or with added 15 mg/L of β-glucan, respectively and 19 and 18 % without or with added 30 mg/L of β-glucan, respectively (Figure 1). In comparison to the oral phase, the recovery increased significantly (p<0.05)) after the gastric phase to 38 and 29% without or with added 15 mg/L of β-glucan, respectively, and to 36 and 37% without or with added 30 mg/L of β-glucan, respectively. Further increase was observed after the intestinal phase to 47 and 36% without or with added 15 mg/L of β-glucan, respectively, and to 45 and 45% without or with added 30 mg/L of β-glucan, respectively. The percentage recovery of individual flavonols also increased through oral, gastric, and intestinal digestion, which was significant in most cases (Table 1). A lower pH and a longer period of digestion over the gastric phase in comparison to the oral phase, might have affected the increased quantity of flavonols. The increase in the intestinal phase might be the result of further release of flavonols entrapped in the matrix, as suggested for flavonols from jaboticaba fruit peel [Quatrin et al., 2020]. The continuous increase of flavonols through three phases of digestion also suggests their stability in the conditions of the digestive tract, which agrees with the study of Bouayed et al. [2012]. However, some studies reported the decrease of flavonols after the digestion of chokeberry juice [Bermúdez-Soto et al., 2007] or apples [Fernández-Jalao et al., 2020]. Furthermore, a de-glycosylation of quercetin glycosides was suggested [Fernández Jalao et al., 2020] since quercetin was found after digestion. Our study supports the resistance of flavonols to hydrolysis/ degradation in the digestive tract. Recoveries of total flavonols in this study were lower than the recoveries after the digestion of apples (84, 84 and 62% after oral, gastric and intestinal digestion, respectively) [Fernández-Jalao et al., 2020].
Anthocyanins
Anthocyanins were released in the oral phase of digestion conducted without or with added 15 or 30 mg/L of β-glucan and they were recovered in 17 and 15% without or with added 15 mg/L of β-glucan, respectively, and 21 and 20% without or with added 30 mg/L of β-glucan, respectively (Figure 1). The recovery increased significantly (p<0.05)) in the gastric phase to 33 and 24% without or with added 15 mg/L of β-glucan, respectively, and to 34 and 33% without or with added 30 mg/L of β-glucan, respectively. Finally, anthocyanin release decreased significantly (p<0.05)) after the intestinal phase (recovery decreased to 9 and 5% without or with added 15 mg/L of β-glucan, respectively, and to 6 and 5%, without or with added 30 mg/L of β-glucan, respectively). Most of the individual anthocyanins showed the same trend as total anthocyanins, the release increased from the oral phase to the end of the gastric phase, and decreased at the end of the intestinal phase (Table 1). The increase of recovery in the gastric phase can be explained by a longer digestion time. However, anthocyanins are also sensitive to pH change. At lower pH, such as the pH in the human stomach, anthocyanins can be released in the form of a flavylium cation, which explains the high quantities after the gastric phase. In the intestine, at higher pH, the anthocyanin chemical structure changes to quinonoidal anions, which will be explained further in the text. This might be the reason for the low quantities at the end of the intestinal phase. A similar decrease of anthocyanins in the intestinal phase has been shown after the digestion of apples [Bouayed et al., 2011], chokeberry juice [Bermúdez-Soto et al., 2007; Yu et al., 2021], polyphenol-rich juice [Stübler et al., 2020] or jaboticaba fruit peel [Quatrin et al., 2020].
Phenolic acids
Phenolic acids showed similar behavior as anthocyanins. After the oral phase conducted without or with added β-glucan where phenolic acids were recovered in 37 and 62% without or with added 15 mg/L of β-glucan, respectively, and 45 and 44% without or with added 30 mg/L of β-glucan, respectively, the recovery significantly increased in the gastric phase in most cases to 75 and 60% without or with added 15 mg/L of β-glucan, respectively, and to 62 and 62% without or with added 30 mg/L of β-glucan, respectively, and then significantly decreased in the intestinal phase in most cases to 59 and 47% without or with added 15 mg/L of β-glucan, respectively, and to 47 and 44% without or with added 30 mg/L of β-glucan, respectively (Figure 1). At low pH, phenolic acids exist mostly in protonated, non-ionic forms. The low pH of the gastric phase favors non-ionic forms of phenolic acids and could have affected the quantities of the recovered total phenolic acids to increase in the gastric phase. At high pH, such as the pH of the intestine, phenolic acids dissociate, and the ratio of dissociated to non-dissociated phenolic acids becomes high. Phenolic acids in the non-ionic form at the end of the intestinal phase could be lower than in the oral or gastric phase, which can explain their decrease in the intestinal phase. Previous studies showed various trends [Bermúdez-Soto et al., 2007; Fernández-Jalao et al., 2020]. Total phenolic acids were stable in the gastric and intestinal digestion of chokeberry [Bermúdez-Soto et al., 2007]; however, one study reported their decrease after the digestion of apples [Fernández-Jalao et al., 2020]. Total hydroxycinnamic acids from apples recovered 63, 53 and 40% after oral, gastric and intestinal digestion, respectively [Fernández-Jalao et al., 2020] or 84–109% and 32–57% after gastric and intestinal digestion [Bouayed et al., 2012]. This is comparable to the results of this study.
Zeta potential
The zeta potential was determined to show the surface charge density of the aronia extract, β-glucan, and a mixture of aronia extract – β-glucan, which helps understand the behavior of phenolics and β-glucan during simulated digestion, and the bonding between them at different pH values. For the aronia extract, it changed from the net positive charge at pH 1.5 (30.1 mV) to the net negative charge at pH 3.0 (–14.5 mV) and pH 7.0 (–15.9 mV) (Table 2). Phenolics present in aronia can contribute to the charge of the aronia extract. At pH 1.5, anthocyanins exist predominantly in the positively-charged flavylium cation form, their structure transforms and at pH 3.0 hemiacetal and chalcone forms exist together with flavylium cations. At pH 7.0, anthocyanins are mostly transformed to negatively-charged quinonoidal anions. Furthermore, phenolic acids exist as non-dissociated forms at pH 1.5. At higher pH, dissociation takes place and the dissociated forms are negatively charged. It is suggested that the aronia extract had a net positive charge at pH 1.5 due to the positively-charged flavylium cations of anthocyanins. At pH 3.0, dissociated forms of phenolic acids might contribute to the net negative charge, and at pH 7.0, dissociated forms of phenolic acids and quinonoidal anions of anthocyanins might contribute to the negative charge of the aronia extract. The net charge of aronia extract confirmed the changes in the structures of anthocyanins and phenolic acids suggested for the intestinal digestion at pH 7.0, which caused the decreased recoveries. But those suggestions need to be confirmed in additional studies. The charge of β-glucan also changed from the net positive charge at pH 1.5 (8.5 and 3.2 mV for 15 and 30 mg/L, respectively) and pH 3.0 (1.6 and 1.3 mV for 15 and 30 mg/L, respectively) to the net negative charge at pH 7.0 (–5.3 and –2.1 mV for 15 and 30 mg/L, respectively) (Table 2). These values are still showing a system that was unstable with not enough charge to prevent repulsion between molecules, which allowed the aggregation of β-glucan particles. Considering the surface charge density of aronia phenolics and β-glucan, reaction between them could take place. At pH 3.0, which is the pH of the gastric simulated digestion, reaction could take place due to hydrogen bonds between OH groups of anthocyanins, phenolic acids, flavonols, and β-glucan, as suggested by Gao et al.[2012], but a positive charge of β-glucan and a negative charge of aronia phenolics could contribute to the bonding due to electrostatic forces. At pH 7.0, which is the pH of the simulated intestinal digestion, β-glucan and aronia phenolics both showed a negative charge, which could cause the repulsion forces and lower the possibility of further reactions. The mixture of the aronia extract and β-glucan showed a net positive charge at pH 1.5 (9.6 and 6.5 mV for 15 and 30 mg/L, respectively), and net negative charges at pH 3.0 (–1.5 and –2.2 mV for 15 and 30 mg/L) and pH 7.0 (–2.0 and –1.1 mV for 15 and 30 mg/L, respectively) (Table 2). The negative charge at pH 3.0 and 7.0 could be a result of negatively-charged phenolics and β-glucan.
Table 2
Zeta potential (mV) of the aronia extract, β-glucan, and a mixture of aronia extract and β-glucan at different pH values.
Digestion with or without β-glucan
Principal component analysis (PCA) on the recovery of phenolics of individual subclasses in each digestion step was conducted to establish the difference between the release of phenolics in the digestion without or with β-glucan (Figure 2). The PCA showed the separation based on β-glucan present or absent in the digestion, which suggests that β-glucan affects the release of phenolics. The first principal component separated phenolics according to the β-glucan presence in the digestion and represented 60.4% of the data variability.
Figure 2
The principal component analysis of phenolics of individual subclasses (flavonols, anthocyanins, and phenolic acids, mg/kg) recovered in the oral, gastric, and intestinal phase of digestion conducted without or with β-glucan (15 and 30 mg/L).
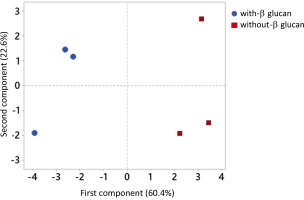
Furthermore, the quantities of recovered phenolics of individual subclasses and total phenolics were compared according to the presence or absence of β-glucan during digestion (Figure 3). Significantly (p<0.05)) lower recoveries of the phenolics of individual subclasses and total phenolics were determined in the gastric and intestinal phases of digestion conducted with the lower concentration of β-glucan, i.e., 15 mg/L, compared to process without β-glucan (Figure 3A). On the other hand, there were no significant differences (p≥0.05) in the recovery of phenolics in the digestion of aronia without β-glucan and with β-glucan at a concentration of 30 mg/L (Figure 3B). This suggests that β-glucan in the low concentration had a stronger effect in entrapping phenolics. Greater contact area between β-glucan and phenolics when β-glucan was present in the lower concentration (15 mg/L) could be the reason for greater entrapment of phenolics.
Figure 3
The quantity of recovered phenolics of individual subclasses from aronia (mg/kg fresh weight, FW) in each digestion step A) without and with 15 mg/L of β-glucan, B) without and with 30 mg/L of β-glucan. The significant differences between quantities with or without β-glucan according to post-hoc Tukey test (p<0.05) are marked by different letters above bars. The data (mean and standard deviation) was obtained in three independent experiments, each measured once (n=3).
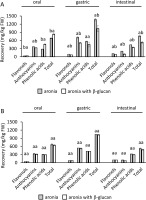
Lower concentrations of phenolics released in the digestion conducted with β-glucan, even though not always statistically significant, suggest there were interactions between phenolics and β-glucan. Interactions resulted in the entrapment of phenolics in the structure of β-glucan, and in the lower concentrations of free phenolics. Studies have shown that phenolics could bond to β-glucans [Gao et al., 2012], which can result in their low concentrations [Larsen et al., 2019; Tomas et al., 2020]. After the simulated digestion of blackberry phenolics, inulin, and pectin acted as an entrapping matrix and lowered the concentration of flavonoids at the end of intestinal digestion [Tomas et al., 2020]. Larsen et al. [2019] also suggested that anthocyanins can form complexes with original and modified pectins, which lowers the concentration of free anthocyanins after short- and long-term incubation.
Enhanced entrapment of phenolics by dietary fibers in the small intestine could result in higher quantities of phenolics carried by fibers to the lower parts of the digestive tract where dietary fibers ferment and phenolics can be released [Solari-Godiño et al., 2017]. In the large intestine, phenolics reveal potentially positive activities, like activities against colon cancer [Wu et al., 2021]. But according to the results of this investigation, the quantity of dietary fibers could be an important factor in the beneficial effects since only β-glucan in the lower concentration showed statistically significant interactions with phenolics.
Adsorption
Comparing the adsorption with low (15 mg/L) and high (30 mg/L) β-glucan concentration, β-glucan adsorbed more phenolics per gram when its concentration was low (15 mg/L) (Table 3). This agrees with the simulated digestion process in which lower quantities of β-glucan resulted in its interaction with phenolics, which lead to statistically significant lower quantities of the recovered phenolics at the end of the digestion process (Figure 3). At a low β-glucan concentration, there might be more chances of interactions between β-glucan and phenolics and larger surface area to which phenolics can adsorb, which leads to more phenolics being adsorbed per gram of β-glucan. At high β-glucan concentrations, there might be more interactions between β-glucan molecules, which might prevent the adsorption of phenolics. Furthermore, the viscosity of β-glucan solutions in high concentrations might prevent phenolic molecules to adsorb.
Table 3
Adsorption capacity of β-glucan for aronia phenolics (qe, mg/g of β-glucan), at different pH values and different β-glucan concentrations.
CONCLUSIONS
This study reports for the first-time the results on the simulated digestion of aronia phenolics with β-glucan. Aronia phenolics were recovered in the digestion process in a lower concentration than in the fruit before the digestion, indicating that some of the phenolics degrade during digestion. Different phases of digestion affected the release of total phenolics. In the oral and gastric phases, the recovery of total phenolics was gradual, and increased from oral to the end of the gastric phase. However, in the intestinal phase, the recovery of total phenolics decreased. Furthermore, the release of phenolics of individual subclasses was different in the three studied digestion phases. Flavonol recoveries increased from oral to gastric and intestinal phases, which might mean a high stability in the digestive tract. After the increase of total anthocyanins and phenolic acids in the gastric phase, the recoveries decreased after the intestinal phase. It can be suggested that the changes of chemical structures of phenolic acids and anthocyanins in the stomach and small intestine, could have affected their recovery, but this is not verified and still needs to be addressed in additional studies. β-Glucan did affect the release of phenolics. The presence of β-glucan (15 mg/L) significantly decreased the quantities of released phenolics at the end of the gastric and intestinal phases. However, with 30 mg/L of β-glucan, a similar but non-significant decrease was determined after oral, gastric, and intestinal digestion. The decrease could be explained by the interactions between β-glucan and phenolics, their entrapment by β-glucan, which finally caused their decreased release in the intestine. The concentration of β-glucan might be a significant factor in the entrapment of phenolics as its lower amounts seem to entrap significant amounts of phenolics.