INTRODUCTION
Authentication of cold-pressed oils can be carried out by means of adulteration detection and quality assessment. Edible oils are susceptible to adulteration with lower-quality oils or substances, which can have a detrimental effect on their nutritional value, safety, and sensory properties [Islam et al., 2022]. Authenticity analysis employs a combination of chemical and physical tests to determine the composition, purity, and quality of the oil. The authenticity analysis becomes increasingly important in the case of the high-value oils, like cold-pressed oils (e.g., flaxseed oil, camelina seed oil, hemp seed oil, olive oil, and avocado oil), which are commonly targeted by fraudulent practices, including adulteration with other substances or blending with cheaper oils [De Souza et al., 2015; Jović & Jović, 2017; Nikolaichuk et al., 2022; Van Wetten et al., 2015; Yanty et al., 2011]. Refined oils, characterized by a neutral flavor, high smoke point and longer shelf life, have been one of the most commonly used and cheapest oils. Therefore, it is also crucial to know the characteristics of widely consumed refined oils (e.g., rapeseed, soybean, sunflower oils), since they can be used as adulterants.
Several factors should be considered when evaluating the authenticity of cold-pressed and refined oils, for instance, the oil’s origin can provide insights into its authenticity [Karim et al., 2015; Piravi-vanak et al., 2022; Rajagukguk et al., 2022; Zhang et al., 2011]. Cold-pressed oils are usually extracted from high-quality seeds or nuts, while refined oils may be sourced from raw materials of average quality. Furthermore, the oil’s color and clarity can be crucial in authenticity assessment. Cold-pressed oils tend to have a darker and cloudier appearance, whereas refined oils have a lighter color due to the elimination of impurities and color pigments during the refining process [Aydeniz Güneser et al., 2017; Gharby, 2022; Vaisali et al., 2015]. The authenticity of an oil can be inferred from its chemical composition. Cold-pressed oils, for instance, may contain more antioxidants and other beneficial compounds than refined oils [Gogolewski et al., 2000; Wroniak et al., 2008].
The analysis of the authenticity of cold-pressed and refined oils usually entails a list of physical, chemical, and sensory tests. Several analytical techniques are employed for this purpose, e.g., gas chromatography (GC) [Xu et al., 2015], high-performance liquid chromatography (HPLC) [Ratusz et al., 2018], and various spectroscopy methods such as UV-visible spectroscopy [Karbasian et al., 2015], nuclear magnetic resonance (NMR) [Siudem et al., 2019], and Fourier transform infrared spectroscopy (FTIR) [Moigradean et al., 2015] being the most widely deployed. However, thermal analysis is an emerging technique that has proved useful in authenticating oil products. Differential scanning calorimetry (DSC) is a thermal analysis technique that measures the amount of heat absorbed or released by a sample as it is heated or cooled, which is often used to analyze oil melting behavior and thermal stability. This information can provide insight into the oil’s purity and authenticity, as well as its thermal stability. Different exothermic or endothermic curves obtained by DSC technique can provide information about the energy changes that occur inside fats or oils during phase transitions, such as melting [Islam et al., 2023] or crystallization [Brożek et al., 2022]. These curves are determined by the structure and behavior of triacylglycerols (TAG), which are the major constituents of fats and oils. Assessment of TAG components showed their influence on polymorphic behavior during phase transition, which affects unique DSC curves [Rousseau et al., 2005; Sato et al., 2013]. Furthermore, degree of unsaturation, indicated by the number of double bonds in the fatty acid chains, influences the energy required for crystallization or melting phase transition [Zhang et al., 2022]. Thus, any added adulterants, such as low-quality oils or other substances, can affect the oil’s thermal properties and can be detected through thermal analysis. In brief, if an oil sample has been adulterated with a lower-grade oil or a non-food-grade substance, the melting and solidification points of the sample will be different from those of the pure oil, and this difference can be detected using DSC [Angiuli et al., 2009; Marikkar, 2014; Dyszel & Baish, 1992; Rudakov et al., 2021].
The aim of this study was to use the whole thermal profiles of melting phase transition of selected edible oils (both cold-pressed and refined oils) for discrimination purposes. A novel approach was taken to combining an untargeted method of DSC with chemometrics in order to distinguish three cold-pressed oils, i.e., flaxseed, camelina, and hempseed oils from such refined oils as rapeseed, sunflower, and soybean oil. Multivariate data analysis tools, i.e., principal component analysis (PCA) and orthogonal partial least squares-discriminant analysis (OPLS-DA), were used to investigate a dataset with a large number of variables. The originality of this study lies in the fact that chemometric methods (PCA, OPLS-DA) were employed for the first time to analyze the whole thermal spectrum of melting phase transition to authenticate cold-pressed oils. To the best of the authors’ knowledge, the use of untargeted analysis of the whole DSC spectrum for the authentication of edible oils has never been reported and is yet to be fully explored.
MATERIALS AND METHODS
Materials
For the experiment, primarily 15 kg of seeds of each cultivar or batch of flax, camelina and hemp were obtained from different sources and then cold-pressed to obtain oils. In the case of flax (Linum usitatissimum L.), seeds of Bukoz cultivar from the Polish Institute of Natural Fibers and Medicinal Plants (Poznań, Poland), Dolguniec cultivar from SEMCO manufactory (Śmiłowo, Poland), Szafir cultivar from two different suppliers, i.e., from SEMCO manufactory and Hodowla Roślin Strzelce Sp. z o.o. (Strzelce, Poland) and seeds of unknown variety from VitaCorn company (Poznań, Poland) were collected. All hemp (Cannabis sativa L.) seeds of the Henola cultivar originated from five different suppliers and were collected from the Polish Institute of Natural Fibers and Medicinal Plants. Three cultivars of camelina (Camelina sativa) seeds originated from five suppliers; seeds of a spring Omega cultivar were purchased from the Poznań University of Life Sciences (Agriculture Research Station Dłoń, Miejska Górka); two camelina seed cultivars (Luna and Śmiłowska) were collected from SEMCO manufactory, which purchased seeds from different suppliers: Luna - a winter variety from two different suppliers and Śmiłowska - a spring variety from two different suppliers. All seeds were pressed in the SEMCO manufactory to obtain the oils at the same conditions, i.e., keeping the temperature below 50°C. The pressed oils were left for 24 h for decantation and stored in brown glass bottles. A total of nine refined oils (three for each type of oil: rapeseed, sunflower, and soybean) were purchased from local Polish markets.
Melting phase transition analysis by differential scanning calorimetry
Melting phase transition analysis of oils was carried out according to the method used for butterfat [Tomaszewska-Gras, 2016] with some modifications, using a DSC 8500 Perkin Elmer differential scanning calorimeter (Waltham, MA, USA), equipped with an Intracooler II and running with Pyris software. Nitrogen (99.999% purity) was the purge gas. Oils (6-7 mg) were weighed into 20-μL aluminum pans (Perkin Elmer, No. 0219-0062) and hermetically sealed. The reference was an empty, hermetically sealed aluminum pan. Analysis started with the oil sample being cooled at a scanning rate of 2°C/min from a temperature of 30°C to −65°C, after which it was heated at a scanning rate of 5°C/min from −65°C to 30°C. For each measurement at a given scanning rate, the calibration procedure was completed with the correct scanning rate. After the analysis, the DSC files were converted to the ASCII format and then analyzed using Origin Pro software, version 2023 (OriginLab Corporation, Northampton, MA, USA). All curves were normalized and the baseline was subtracted to project the DSC curves of all investigated samples in the same scale. Peak temperature (T, °C), peak heat flow (h, W/g), and enthalpy (∆H, J/g) were measured from the melting curves. Peak temperature was determined at the maximum heat flow on the curve for the selected peak. Peak heat flow was established as the maximum value of heat flow for the normalized peaks. Enthalpy was determined by integrating the area under the curve of the heat flow (J/s) vs. temperature (°C). The number of analytical repetitions was two for each oil sample. Therefore, for each cold-pressed oils, five samples were analyzed in duplicate, and for each refined oils, three samples were analyzed in duplicate.
Statistical analysis
Mean and standard deviation were calculated in order to present results that were obtained from ten measurements (n=10) for each cold-pressed oil and from six measurements (n=6) for each refined oil. The SIMCA software version 16.1 (Sartorius Stedim Data Analytics AB, Umea, Sweden) was used to conduct multivariate data analysis, i.e., PCA and OPLS-DA. Both PCA and OPLS-DA models were cross-validated, and the OPLS-DA models were also validated using permutation testing. For these models, X represents the normalized heat flow data matrix obtained from 7471 variables, Y represents the types of oils, and the predictive variables in X were used to classify and predict the oil types. To assess the quality of the models, the model statistics parameters: R2X(cum), R2(cum), R2Y(cum), Q2(cum), were obtained through cross-validation. Model performance was evaluated by examining the explained variation R2(cum), which represents the goodness of fit for X- and Y-variables, and the predictive variation Q2(cum), which represents the goodness of prediction for the fit of predicted variables.
RESULTS AND DISCUSSION
DSC melting profiles of cold-pressed and refined oils
Cold-pressed (flaxseed, camelina seed and hempseed) and refined (rapeseed, soybean and sunflower seed) oils were analyzed for phase transition during heating to establish their melting profile by considering the full spectrum of the DSC curves as fingerprint of the oil. DSC is a highly regarded thermal analysis technique that can explain various properties of these oils, such as their phase transition temperature and magnitude of the thermal effect (heat flow) during individual processes of transition, and enthalpy as energy changes involved in the process. Figure 1 presents the melting curves of cold-pressed oils. To plot the melting curves, all those oils were subjected to crystallization before starting the heating program. The formation of peaks during the melting phase transition is a manifestation of the energy required to overcome the intermolecular forces holding the crystals together, which is absorbed by the sample during heating, resulting in endothermic peaks. As can be seen from Figures 1A and 1C, four peaks appeared on the melting curves, i.e., at −36°C, −30°C, −25°C and −13°C for flaxseed oil; and at −41°C, −32°C, −24°C and −17°C for hempseed oil. In turn, the melting profiles of seed oils of all camelina cultivars were definitely different compared to the profiles of flaxseed and hempseed oils. The second peak was manifested as an exothermic thermal event at −34°C, whereas the other two peaks at −38°C and −12°C were clearly endothermic in nature (Figure 1B). The exothermic peak appeared as a downward deflection in the baseline of the DSC curve of camelina seed oil, which indicates that the sample is undergoing a polymorphic transition by recrystallization. The presence of an exothermic peak during the melting phase transition of camelina seed oil was also reported by other authors [Rudakov et al., 2021]. Recrystallization of the polymorphic crystals of triacylglycerols into a more stable form was observed for flaxseed, hempseed and canola oil, especially at lower heating rates, i.e., 1°C/min [Teh & Birch, 2013]. Although seeds of different plant cultivars were analyzed in the experiment, melting curves showed similarities between the samples of individual oil types, thus unique thermal profiles were obtained for each oil type. The melting process of flaxseed oils of all cultivars started at the temperature around −48°C, after which the initiation of the first peak occurred. The second peak appeared at around −30°C, which is the major peak of the transition, with two more shoulder peaks as the third and fourth peak at the end of transition (at temperatures of −25 and −13°C, respectively). For camelina and hempseed oils, the onset of the melting process was earlier than for flaxseed oils (for both at around −52°C). Although the curves for hempseed oils show the same number of endothermic peaks as for flaxseed oils (four peaks), their melting profile was noticeably different from that of flaxseed oils.
Figure 1
Differential scanning calorimetry (DSC) melting curves of cold-pressed oils at a scanning rate 5°C/min; (A) flaxseed oils; (B) camelina seed oils; (C) hempseed oils. Different color curves represent oil samples from different suppliers.
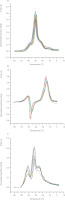
The behavior of oils during the phase transitions was quantified by the thermodynamic parameters, such as peak temperature, peak heat flow and enthalpy. Results are presented in Table 1. These parameters can be substantial for the authenticity assessment of oils. The peak temperatures are presented as T1, T2, T3 and T4 from the first to the fourth peak appearing while oil crystals were melted. The magnitude of transition measured as peak heat flow was also calculated for all peaks after the normalization of the melting curve. A normalized heat flow of the major peak (h2) for flaxseed oil was higher (0.49 W/g) than that of the major peak (h2) for hempseed oil (0.35 W/g). However, the greatest magnitude of transition was observed for the fourth peak (h4) of camelina oils (0.70 W/g). The first and third peak identified for hempseed oil had the higher heat flows (0.20 and 0.19 W/g, respectively) than those of flaxseed oils (0.13 and 0.14 W/g, respectively). For flaxseed and hempseed oil, the major peak (h2) appeared when the peak temperature reached approximately −30°C and −32°C, whereas for camelina oil the main peak was observed at the end of the melting transition when peak temperature reached approximately −12°C. In turn, the exothermic peak of camelina oil had peak heat flow minimum (h2) of −0.40 W/g. Enthalpy can provide insight into the degree of crystallinity or molecular weight, or amorphousness of the samples. Amongst the cold-pressed oils, flaxseed oils showed the lowest ∆H value (62.73 J/g), compared to camelina seed oil (65.09 J/g) and hempseed oil (69.53 J/g). Other authors determined the melting enthalpy for different transformants of camelina oil in the range from 42 to 57 J/g [Rodríguez-Rodríguez et al., 2021] and for flaxseed oil from 54 to 62 J/g [Punia et al., 2020; Zhang et al., 2011].
Table 1
Differential scanning calorimetry (DSC) parameters of the melting curves of cold-pressed oils.
[i] All values are mean ± standard deviation (n=10); T1, T2, T3 and T4 represent the first, second, third and fourth peak temperatures, respectively; h1, h2, h3 and h4 mean heat flow for the first, second, third and fourth peak, respectively; ∆H represents enthalpy for the whole melting phase transition.
The melting profiles of flaxseed and camelina seed oils in our study are consistent with the results obtained by Rudakov et al.[2021] who used DSC at 5°C/min heating rate. These authors mentioned that the characteristics of the melting curves depended on the triacylglycerol (TAG) profile of the oil samples, which causes differences in the number of peaks, peak temperature values, and the magnitude and temperature ranges of melting transition, as well as enthalpy of transition. Similarly to this study, they also observed four endothermic peaks appearing for flaxseed oils, and three peaks for camelina seed oils (where the second peak was exothermic). Similar four peaks at −38, −31, −24, −13°C were identified by Zhang et al. [2014] for the melting profiles of flaxseed oil obtained with the same heating rate of 5°C/min. Our previous study showed that the melting profiles of oils were very strongly influenced by the scanning rate used for heating [Islam et al., 2023; Tomaszewska-Gras et al., 2021]. In turn, Teh & Birch [2013] compared the melting profiles of cold-pressed flaxseed and hempseed oils at a lower heating rate of 1°C/min, for which they determined two endothermic peaks at −40°C and −18°C for hempseed oil and at −36°C and −15°C for flaxseed oil, as well as one exothermic peak at −39°C for hempseed and at −30°C for flaxseed oils. They suggested that the polymorphic transitions indicating the recrystallization of unstable structures were due to the low heating rate. Another study, carried out with a higher scanning rate of 10°C/min, showed that flaxseed hull oils exhibited three peaks of the melting transitions at −33°C, −25°C and −14°C [Oomah & Sitter, 2009], which indicates that a higher heating rate may reduce peak resolution.
Authentication of cold-pressed oils requires knowledge and verification of the characteristics of oils which can be used as adulterants. These most often used ones include refined oils, because they are colorless and odorless, but also because they are cheap and widely available. The melting characteristics of refined rapeseed, soybean and sunflower oils are presented in Figure 2. The melting curves of rapeseed oils were characterized by two endothermic peaks, where the second peak was the major peak, appearing at −13°C, while the melting temperature range was from −30°C to −5°C. In the case of DSC profiles of soybean and sunflower oils, four and three endothermic peaks were identified, respectively, within the transition temperature range from −45°C to 0°C for both oils. For soybean and sunflower oils, the main peak was at a similar temperature, around −24°C. However, there were two peaks (at −18°C and −5°C) after the main peak for soybean oil and one peak at −9°C for sunflower oil. For soybean and sunflower oils, the curves showed differences in the number of peaks; however, corresponding peaks had similar magnitudes of transition and there was a similar temperature range of melting transition. These can be more explicitly described by analyzing the results shown in Table 2 collating DSC parameters, like peak temperature, peak heat flow and enthalpy of the refined oils. The highest transition magnitude was observed for the second peak (h2) of the rapeseed oil samples, i.e., 0.48 W/g, whereas the main peak heat flows of the soybean oils and sunflower oils were 0.28 and 0.31 W/g, respectively. Additionally, during melting transition, two more shoulder peaks were detected for soybean oils, and one for sunflower oils. Comparing ∆H determined for the whole melting process, soybean oils show the lowest value (58.42 J/g), in contrast to rapeseed oils, for which the highest enthalpy was measured (63.91 J/g). A similar experimental approach was presented by other authors [Tan & Che Man, 2000], who characterized canola, soybean and sunflower oils after heating at a rate of 5°C/min and identified two peaks for canola and four peaks for the soybean and sunflower oils, where the position of peaks differed from that found in our study. Comparable results obtained for the melting profiles at a heating rate of 5°C/min for sunflower oils with three peaks (−36°C, −27°C, and −11°C) and rapeseed oil with two peaks (−23°C and −15°C) were reported by Rudakov et al. [2021]. Other authors [Teh & Birch, 2013] reported two major endothermic peaks at −23°C and −9°C for cold-pressed canola oils with a 1°C/min program, which is comparable to the results of our study.
Figure 2
Differential scanning calorimetry (DSC) melting curves of refined oils at a scanning rate 5°C/min; (A) rapeseed oils; (B) soybean oils; (C) sunflower seed oils. Different color curves represent oil samples from different suppliers.
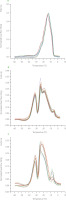
Table 2
Differential scanning calorimetry (DSC) parameters of the melting curves of refined oils.
[i] All values are mean ± standard deviation (n=6); T1, T2, T3 and T4 represent the first, second, third and fourth peak temperatures, respectively; h1, h2, h3 and h4 mean heat flow for the first, second, third and fourth peak, respectively; ∆H represents enthalpy for the whole melting phase transition.
Chemometric analysis of melting profiles of cold-pressed and refined oils
The main goal of this study was to use data obtained from the DSC technique to authenticate three cold-pressed oils and to differentiate them from the refined oils by analyzing the melting phase transitions. Since it could be seen (Figure 1 and 2) that the DSC profiles obtained for all oils (cold-pressed and refined) were visually different, it was necessary to decide at this stage which results and methods to choose for discrimination. To solve this problem, two potential approaches were considered in this study. The first was to compare the parameters determined from the curves, which are presented in Table 1 and Table 2. The second approach was based on comparing the entire phase transition spectrum using chemometric methods. The disadvantage of the first approach was the fact that melting profiles consist of a different number of peaks at different positions, which makes it difficult to compare them with each other. Thus, the authors decided to compare the whole melting profiles of oils expressed by normalized heat flow (W/g), which were tested as variables using SIMCA software. Multivariate data analyses, i.e., PCA and OPLS-DA, were conducted to assess the usability of DSC melting profiles in classifying and distinguishing cold-pressed and refined oils. The first step was to compare two groups of oils, refined oils vs. cold-pressed oils. PCA - a widely used, unsupervised analysis - was employed to uncover and visualize the underlying patterns of variations within a dataset. Figure 3A shows a score plot with oils separated into two groups: refined and cold-pressed ones. Based on the DSC data matrix, the two principal components, t[1] and t[2] were established, which accounted for 80.8% of the variation in the values of normalized heat flow of the melting profiles. Next, an OPLS-DA model was built on the same data matrix, which focused on the separation of two oil classes: refined and cold-pressed ones (Figure 3B). The OPLS-DA is a multivariate statistical method that is commonly used for the classification and prediction of data with multiple variables. The OPLS-DA model separates the systematic variation in X (normalized heat flow) into two parts: one that is linearly related (and therefore predictive) to Y (representing classes), and one that is orthogonal to Y. The Y-predictive part represents the between-class variation and the Y-orthogonal part constitutes the within-class variation. A score plot of cold-pressed and refined oils classified into six classes in the space of two components: t[1] - predictive and to[1] - orthogonal, is shown in Figure 3B. The model fit was described by the coefficient R2X(cum)=0.807, which is the cumulative R2X of the fractions of the X variation modeled in the component, using the X model, the R2(cum)=0.978, which is the cumulative R2 of the fractions of Y variation modeled in the component, using the X model, and Q2(cum)=0.977, which is cumulative Q2 of fractions of Y variation predicted according to cross-validation in the component, using the X model. It can also be noticed that the separation in both classes, occurring relative to components t[1] and t[2], is additionally connected with the appearance of two subclasses for each class, which are located on opposite sides (Figure 3A). However, the difference between subclasses is smaller for refined oils compared to cold-press oils. This suggests some similarities inside both classes, leading to their differentiation. The existence of subclasses inside each class was confirmed by the OPLS-DA method, where orthogonal component to[1] also shows differentiation inside the class of unrefined oils (Figure 3B).
Figure 3
Differentiation between cold-pressed and refined oils based on their differential scanning calorimetry (DSC) melting profiles; (A) principal component analysis (PCA) score plot; where t[1] and t[2] are the first and second principal components, respectively; (B) orthogonal partial least square-discriminant analysis (OPLS-DA) score plot; where t[1] and to[1] are predictive and orthogonal components, respectively.
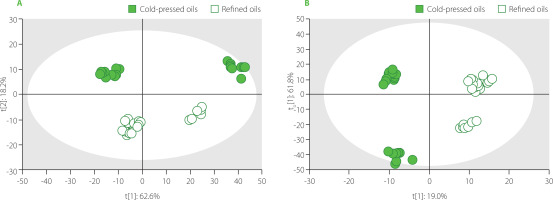
Since the orthogonal component in X was significant (R2X = 0.618), indicating that the variation within the class of cold-pressed oils and within the class of refined oils was high (Figure 3B), the next step was to analyze all six types of oils as six classes by using PCA (Figure 4A) and OPLS-DA (Figure 4B). Figure 4A shows a score plot of the PCA results with the oils separated into six groups: three refined oils (rapeseed, soybean and sunflower) and three cold-pressed oils (flaxseed, camelina, hempseed), in the space of the two first principal components t[1] and t[2], which accounted for 80.8% of the total variation. The score plot with the clear classification and discrimination of six types of oils conducted by means of the OPLS-DA is shown in Figure 4B. Five predictive components (P1-P5) and two orthogonal components in X (O1, O2) were calculated by the OPLS-DA. The variation modelled for X using all predictive components and orthogonal components in X gave the value of R2X(cum)=0.971, which is a measure of model fit. The next parameters of OPLS-DA, R2(cum) and Q2X(cum), were equal to 0.916 and 0.887, respectively. Individual values of Q2 calculated for five components are the fractions of the Y variation predicted according to cross-validation in the component using the X model. All Q2 values were in the range from 0.143 to 0.198 and all were higher than the limit of 0.01, which is the critical value of Q2 below which the component is insignificant.
Figure 4
Distinguishing oils based on their differential scanning calorimetry (DSC) melting profiles; (A) principal component analysis (PCA) score plot; where t[1] and t[2] are the first and second principal components, respectively; (B) orthogonal partial least square-discriminant analysis (OPLS-DA) score plot; where t[1] and t[2] are predictive and orthogonal components, respectively. CA, camelina seed oil; FL, flaxseed oil; HP, hempseed oil; R, rapeseed oil; SB, soybean oil; SF, sunflower seed oil.
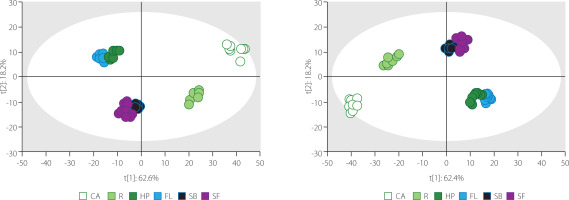
Summing up, it can be stated that all parameters (R2X(cum), R2(cum) and Q2X(cum)) indicated that the model established by the OPLS-DA fitted the data of DSC profiles and enabled reliable classification of oils into six classes. The variables influence on projection (VIP) plot shown in Figure 5 reflects selected variables, which are of the highest importance for oil differentiation. Normalized VIP values were in the range from 0.3 to 1.6, while the average squared VIP value was 1. Since only variables with VIP values above 1 are important, only such variables are shown in Figure 5. It can be seen that, in this model, the most important for oil discrimination were the heat flow values of the DSC profiles measured at temperatures of −29.44±0.32°C, −32.48±1.80°C, −14.09±1.61°C, −9.60±0.71°C, −24.36±0.94°C, and −41.39±0.77°C.
CONCLUSIONS
This study presents a novel approach for authenticating oils by using the whole spectrum of their DSC melting profiles. The DSC profiles combined with advanced chemometric methods of OPLS-DA were used to distinguish cold-pressed oils (flaxseed, camelina, hempseed) from refined oils (rapeseed, sunflower, soybean) in order to establish a model for their classification. The results showed that the DSC melting profiles can be considered as a fingerprint of each oil, since they differed in the number of peaks and their position. Flaxseed and hempseed oils exhibited four endothermic peaks, in contrast to camelina seed oil, for which three peaks with one exothermic event were detected. For the refined oils, two endothermic peaks were detected for rapeseed oil, three for sunflower oil and four for soybean oil. Additionally, it was stated that thermodynamic parameters, such as peak temperature, peak heat flow and enthalpy, differed for each type of oil. The results from the PCA and OPLS-DA showed successful classification of different edible oils into two classes (refined and cold-pressed), as well as into six classes according to the oil type. The model fitted the data well, as indicated by the R2X(cum), R2(cum), and Q2X(cum) values, which assessed the variation in the X (normalized heat flow) and Y (classes) data. Furthermore, it was shown that certain heat flow values measured at specific temperatures were crucial for differentiating the oils. These variables played a significant role in the discrimination of oils based on their melting profiles.
The study provides practical information on the utility and the potential of the DSC profiles for the detection of frauds, as it was in the case of olive oil scandal in Western Europe in 2019, where refined sunflower oil was colored with chlorophylls and beta-carotene to mirror olive oil and sold as extra virgin olive oil. The approach presented in this study could lead to future research addressing more expensive and highly nutritious plant fats and oils available on the market, to build a database of their fingerprints to be analyzed by chemometric methods for authentication.