ABBREVIATIONS
4-HNE, 4-hydroxynonenal; 8-oxo-dG, 7,8-dihydroxy-8-oxo-2′-deoxyguanosine; AAPH, 2,2′-azobis(2-methylpropionamidine) dihydrochloride; ACL, antioxidant capacity in lipids; ACW, antioxidant capacity in water; AGEs, advanced glycation end products; ALEs, advanced lipoxidation end products; AOPPs, advanced oxidation protein products; ATP, adenosine triphosphate; BMI, body mass index; CAT, catalases; CUPRAC, cupric ion reducing antioxidant capacity; DNPH, dinitrophenylhydrazine; ELISA, enzyme-linked immunosorbent assay; ERK, extracellular signalregulated kinase; F2-IsoPs, F2-isoprostanes; Foxo3a, forkhead box O3; GPx, glutathione peroxidase; GR, glutathione reductase; GSH, glutathione; GSK3β, glycogen synthase 3; GSSG, glutathione disulfide; GST, glutathione transferase; HAT, hydrogen atom transfer; HDACs, histone deacetylases; HDL, high-density lipoprotein; HEK293, human embryonic kidney 293; KD, ketogenic diet; LGIT, low glycemic index treatment; MAD, modified Atkins diet; MCT, medium-chain triglycerides; MCTKD, medium-chain triglycerides-based KD; MDA, malonaldehyde; MPO, myeloperoxidase; Mt2, metallothionein-2; NAD+, nicotinamide adenine dinucleotide; NADPH, nicotinamide adenine dinucleotide phosphate; NEAC, nonenzymatic antioxidant capacity; NOX, NADPH oxidases; NQO1, quinone oxidoreductase; Nrf2, NF E2-related factor 2; ORAC, oxygen radical absorbance capacity; OS, oxidative stress; oxLDL, oxidized low-density lipoprotein; PCL, photochemiluminescence; PUFAs, polyunsaturated fatty acids; RNS, reactive nitrogen species; ROS, reactive oxygen species; SCOT, succinyl-CoA: 3-ketoacid CoA-transferase; SET, single electron transfer; SOD, superoxide dismutase; TAC, total antioxidant capacity; TBA, thiobarbituric acid; TBARS, thiobarbituric acid-reactive substances; TRAP, total radical-trapping antioxidant parameter; UCP, uncoupling protein; VLCKD, very low-caloric ketogenic diet; VOCs, volatile organic compounds; XDH, xanthine dehydrogenase; XO, xanthine oxidase.
INTRODUCTION
The interest in the impact of diet on human health has surged over the last few years. Consumers are increasingly mindful of their food choices, seeking ways to enhance their health through the selection of appropriate food products. Furthermore, individuals are paying greater attention to the physiological effects and endeavouring to comprehend the intricate biochemical processes. Consequently, the food industry and numerous scientific groups have developed a growing array of functional foods, novel foods, and bioactive compound-enriched foodstuffs [Moolwong et al., 2023; Nguyen et al., 2023; Ta et al., 2023; Wieczorek et al., 2022]. Additionally, there is a rise in the development of new diets and diversification of applied dietary plans. Various diets have been applied and proposed for weight management [Johnston et al., 2014], of these the ketogenic diet (KD) is currently gaining in popularity [Drabińska et al., 2021].
The increase in the prevalence of noncommunicable diseases observed in recent years can be considered a consequence of a rapid rise in obesity prevalence [WHO, 2016, 2023]. One of the factors contributing to the development of obesity-related consequences is oxidative stress (OS). It can be attributed to cellular damage caused by reactive oxygen species (ROS) combined with the underproduction of antioxidant mechanisms. Moreover, disturbances in redox metabolism can lead to the development of immunological diseases and cancer [Nathan & Cunningham-Bussel, 2013]. Consequently, the significance of OS in the pathogenesis of diseases is garnering attention, focusing on understanding ongoing mechanisms and eventually targeting OS in applied therapies in the future [Nathan & Cunningham-Bussel, 2013].
This review summarizes and discusses existing knowledge about KD and ROS homeostasis. Additionally, it presents the available tools for evaluating OS status, listing their potential and drawbacks. Finally, the review addresses future studies needed to understand the connection between KD and OS.
KETOGENIC DIET
KD is a dietetic regime entailing a high consumption of fat with a low intake of carbohydrates, leading to the ketosis state. It is a diet consisting of various fat sources, such as plant-based oils, nuts, avocado, meat, cheeses, high-fat dairy products and simultaneously limiting carbohydrate sources, such as bakery goods, potatoes and grains. Compared to other low-carbohydrate diets, KD is distinguished by the shift of metabolism into ketosis. The ketosis state is a physiological process, where the concentration of ketone bodies (β-hydroxybutyrate, acetoacetic acid and acetone) in the bloodstream exceeds 0.5 mmol/L with a normal level of glucose and insulin [Paoli, 2014]. In the case of glucose scarcity, ketone bodies are used as a source of energy. From a biochemical point of view, KD mimics the starvation or fasting state, during which the body shifts from glucose as a main source of energy to ketone body utilization and enhanced gluconeogenesis and ketogenesis.
Originally, KD was developed to treat medically intractable epilepsy due to its neuroprotective properties [Ułamek-Kozioł et al., 2019]. The mechanisms of the neuroprotective effects of KD remain unclear; however, a potential mechanism is related to the normalization of energy dysregulation. KD has been found not only to reduce the frequency of seizures in patients with epilepsy [Keene, 2006] but also may help with Alzheimer’s disease [Ota et al., 2019], improve motor functions and cognitive functions in Parkinson’s disease [Phillips et al., 2018], and support other neurodegenerative diseases [Zhao et al., 2006]. Recently, the interest in the applications of KD is wider. Reported health benefits being the consequences of KD include the normalization of glycaemia and insulin sensitivity, mitigating the severity of type 2 diabetes, fast reduction of body weight in the first stage of KD, improved autophagy and many others [Liśkiewicz et al., 2021]. However, to observe the beneficial effects of KD, the adaptation step is required. The adaptation to ketosis usually takes 4–6 weeks and is often characterized by unpleasant symptoms, including headache, lack of energy, irritability, dizziness, constipation, and muscle cramps [Bostock et al., 2020; Harvey et al., 2018]. Since many of these side effects are similar to flu, the common name used by the general public is “keto flu”. These symptoms result from the loss of electrolytes with urine due to lower levels of insulin responsible for the reuptake of mainly sodium and potassium, the dehydration which is typical of all high-fat diets, and the lack of glucose to provide energy when the body still has not shifted into the utilization of ketone bodies [Harvey et al., 2018]. Usually, the symptoms pass after reaching nutritional ketosis and can be reduced by a higher intake of water, medium-chain triglycerides (MCT), and electrolytes. The body needs to prepare for the increased level of ketone bodies, which can enter the non-hepatic cells via monocarboxylic transporters, and it is suggested that the expression of monocarboxylate transporters increases after 4–6 weeks of ketosis [Barry et al., 2018].
It is also worth mentioning that KD is not a suitable diet for everyone as it may be harmful to individuals with liver and kidney diseases, type 1 diabetes, and cardiovascular diseases, although these risks are not sufficiently scientifically proven [Watanabe et al., 2020]. Moreover, it is suggested that since it is a diet limiting the consumption of many food products, long-term adherence to KD may lead to nutritional deficiencies and consequences like anaemia and osteoporosis [Crosby et al., 2021]. Therefore, the appropriate optimization of KD, securing the intake of a sufficient amount of fibre, vitamins, and elements is required.
KD extends into various types, which makes it difficult to compare the existing results. Depending on the fat intake or calorie intake, and consequently the ketosis state, a few types of KD can be distinguished, including classical KD, Atkins diet, modified Atkins diet (MAD), MCT-based KD (MCTKD), low glycemic index treatment (LGIT), and very low-caloric KD (VLCKD) [Barzegar et al., 2021; Moreno et al., 2016]. The most restrictive is the classical KD, where the weight ratio of fat to carbohydrates and proteins together is 4:1, which consists of approx. 90% of fat intake. This diet is used only for the treatment of non-responsive epilepsy and is characterized by low palatability. The Atkins diet was the first approach to apply KD for obesity management. It consists of four phases: (1) induction with limit of 20 g of carbohydrates daily; (2) increase of carbohydrate intake to 25–50 g daily; (3) the consumption of carbohydrates reaches 80 g per day and is followed until the desirable bodyweight is obtained, and (4) up to 100 g of carbohydrates is allowed and is followed to maintain the weight reduction. Notably, only the first one is actually ketogenic. In later steps, the carbohydrate intake is increased preventing ketosis from being maintained. The MAD is a more palatable version of KD. In this diet, only the first phase of the Atkins diet is maintained for the whole time, making this diet more palatable and maintaining the neuroprotective properties of the KD. The application of MCT allows for the further reduction of fat intake without compromising the ketosis state. MCT is the most efficient in ketone body formation and a more accessible form of energy for non-hepatic cells among the fat sources. LGIT is characterized by the lowest proportion of fat (approx. 60–65%) and the consumption of products only with low glycemic index. Finally, VLCKD is a dietetic regime providing only 500–700 kcal daily and is usually applied before bariatric surgery in clinical conditions [Pilone et al., 2018].
The wide array of KD types makes it difficult to clearly conclude the physiological effects of its administration. Importantly, not every study referring to KD measured the state of ketosis, and probably in some studies KD was not actually applied. According to Zilberter & Zilberter [2018], the “ketogenic ratio” they had developed can be calculated to ensure that the diet is actually ketogenic. The authors evaluated 62 clinical trials which reported following the KD, and only 25 had an acceptable ketogenic ratio. Therefore, the lack of the ketosis measurement and insufficient ketogenic ratio may lead to incorrect conclusions from the studies with reported KD.
OXIDATIVE STRESS
Reactive oxygen species (ROS) are highly reactive molecules generated as a by-product of metabolism, which are involved in cell signalling, redox homeostasis and the host defense system. ROS family includes superoxide, hydrogen peroxide, singlet oxygen, organic peroxides, hypohalous acids and ozone (Table 1). Apart from ROS, also other molecules, such as nitrogen species (nitric oxide and nitroxyl anion), hydrogen sulfde and its anion, and carbon monoxide, can be considered as reactive signalling molecules [Nathan & Cunningham-Bussel, 2013]. The sources of ROS can be exogenous (air pollution, γ-irradiation, drugs) or endogenous, such as mitochondrial respiratory chain, nicotinamide adenine dinucleotide phosphate (NADPH) oxidases, lipoxygenases, cyclooxygenases, cytochrome P450 enzymes, metal storage proteins and many others.
Table 1
Reactive species.
Oxidative stress (OS) is a state when the production of ROS is higher that their catabolism [Nathan & Cunningham-Bussel, 2013]. When the ROS concentration is too high, the OS is induced, leading to the development of pathological states [Qu et al., 2021]. The catabolism of ROS is conducted mainly via enzymatic reactions with superoxide dismutases (SOD), catalases (CAT), enzymes of the glutathione redox cycle, thioredoxin reductases, peroxiredoxins and methionine sulfoxide reductases [Nathan & Cunningham-Bussel, 2013]. Moreover, non-enzymatic reactions with ascorbate, pyruvate, α-ketoglutarate, and oxaloacetate can lead to ROS depletion. The body homeostasis is maintained despite the ROS production, and many injuries caused by ROS can be reversed. On the other hand, some oxidative damages, such as carbonylation on the amino acid side of the protein, cannot be reversed and require cell degradation. It is considered that the biological importance of redox processes is equal to phosphorylation-dephosphorylation reactions [Sies et al., 2017].
OS is an important factor in many diseases; however, it remains unclear whether redox imbalance is a cause or the consequence of disease states [Sies et al., 2017]. In obesity, the malfunction of adipose tissue with exceeded energy intake over the energy expenditure triggers metabolic stress, which leads to increased inflammatory responses, which then results in the interrelated complications including glucose intolerance, hypertension, insulin resistance, dyslipidemia, liver diseases, stroke and cancer. OS is linked to impaired insulin signalling. Increased ROS levels can interfere with insulin receptor signalling, promoting insulin resistance in target tissues, such as muscle and adipose tissue. This can lead to reduced glucose uptake by cells and, consequently, glucose intolerance. Moreover, ROS can cause endothelial dysfunction, reducing the bioavailability of nitric oxide. This leads to impaired blood vessel dilation and increased vascular resistance [Ho et al., 2013]. Finally, chronic inflammation and OS can damage DNA, leading to mutations and promoting carcinogenesis [Alhamzah et al., 2023]. OS contributes to a cascade of events that can lead to serious health consequences. Therefore, managing OS is a potential strategy for preventing or mitigating these complications.
OS has been repeatedly suggested to be involved in the progression of neurodegenerative diseases, such as Alzheimer’s disease, Parkinson’s disease and amyotrophic lateral sclerosis. A significant oxidation of lipids, proteins, and DNA is typical of these diseases [Bedard & Krause, 2007]. Such damages have the potential to induce cell death through various mechanisms, either by inhibiting crucial processes or by upregulating toxic cascades.
METHODS OF OXIDATIVE STRESS EVALUATION
The importance of OS in the development of various pathological states in the human body has raised the need for methods allowing the evaluation of its level. The methods aim to assess the biological redox status, the health-beneficial effects of dietary antioxidants, and the progression and risk of disease developments. Since OS is a process involving many metabolic pathways, there is no single marker enabling its level assessment. Therefore, a combination of different markers is analyzed to provide the most comprehensive view of OS. Despite the numerous markers proposed over the last decades, there is a lack of consensus regarding their efficacy, standardization, validation, and reproducibility [Marrocco et al., 2017]. The choice of specific markers should depend on the experimental design, type of samples and the available analytical techniques. Markers with the clinical significance are mainly measured in the blood samples and urine [Jørs et al., 2020], but can also be measured in saliva or exhaled breath [Bartoli et al., 2011; Pelclova et al., 2018]. This section further presents the most commonly used non-invasive markers for clinical trials and discusses their potentials and limitations.
Markers of reactive oxygen species-induced modifications
Long-term exposure to high levels of ROS can lead to structural modifications of cellular compartments, including lipids, proteins, and nucleic acids. This can result in the systemic or tissue-specific formation of by-products, alteration of protein functions, and changes in gene expressions. Based on this, specific markers related to ROS-induced modifications can be defined and summarized in Figure 1.
Figure 1
The most commonly used markers of oxidative stress representing reactive oxygen species (ROS)-induced modifications.
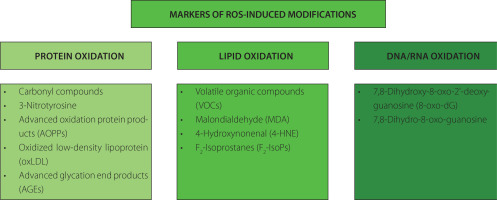
Protein modification products
Proteins including enzymes, may be damaged by OS, leading to conformational modifications that result in a loss or impairment of enzymatic activity [Davies, 2016]. Protein modifications include chain fragmentation, site-specific amino acid modifications, proteolysis susceptibility, changes in the electric charge, and alterations in enzymatic activity. Various amino acid residues can undergo oxidative changes, such as sulfur-containing residues undergoing oxidation, aromatic and aliphatic groups undergoing hydroxylation, tyrosine residues undergoing nitration, cysteine residues undergoing nitrosylation and glutathionylation, aromatic groups and primary amino groups undergoing chlorination, and some amino acid residues being converted to carbonyl derivatives [Davies, 2016]. Moreover, oxidation can lead to the disruption of the polypeptide chain, resulting in conformational changes.
The most widely used markers of oxidative protein damage are carbonyl compounds (aldehydes and ketones) [Marrocco et al., 2017]. Their formation involves the covalent modification of the side chains of specific amino acid, such as lysine, arginine, proline, and threonine. The measurement of carbonyl compounds is usually done via dinitrophenylhydrazine (DNPH) assay, western blotting and mass spectrometry [Kehm et al., 2021].
Another marker of OS is 3-nitrotyrosine, which is the main product of nitration of tyrosine residues in the protein in the presence of reactive nitrogen species (RNS), such as peroxynitrite [Kehm et al., 2021]. During this process, a nitro (–NO2) group is substituted to the phenolic ring of tyrosine. The presence of 3-nitrotyrosine can be detected using techniques such as immunoblotting or mass spectrometry.
The reaction of proteins with chlorinated oxidants can lead to the formation of advanced oxidation protein products (AOPPs), with 3-chloro-tyrosine and 3,5-dichloro-tyrosine as the main examples [Marrocco et al., 2017]. Elevated levels of AOPPs have been detected in the inflammatory diseases, diabetes, and the kidney failure [Cristani et al., 2016; Taylor et al., 2015]. The level of AOPPs is usually measured using the colorimetric techniques.
Oxidized low-density lipoprotein (oxLDL) has also been proposed as a marker of OS and cardiovascular diseases. Nonetheless, employing oxLDL as a biomarker for OS has faced criticism due to the varied nature of oxidation products, the limited specificity of antibodies, and the divergent outcomes observed depending on the deployed assay [Frijhoff et al., 2015; Marrocco et al., 2017]. Moreover, the most commonly used assays to measure oxLDL, detect also the native LDL; therefore, serious doubts have been raised regarding the efficacy of oxLDL as an indicator of OS and its clinical relevance in predicting cardiovascular and related diseases beyond that offered by LDL cholesterol alone.
The formation of advanced glycation end products (AGEs) is often associated with OS and is enhanced by the ROS. Therefore, although it is not a direct marker of OS, it is commonly measured to establish hyperglycemic, hyperlipidemic and OS conditions [Frijhoff et al., 2015]. AGEs are a group of heterogenous compounds formed upon a non-enzymatic reaction between reducing sugars and amino groups of proteins, lipids, or nucleic acids, called Maillard reaction. This process occurs both endogenously (due to aging) and exogenously (AGEs delivered from diet). Accumulation of AGEs can lead to the cross-linking of proteins, modifying their structure and function. This can affect the normal physiological functions of proteins and contribute to aging-related complications. AGEs can be measured using the immunoassays, fluorescence spectroscopy and western blotting [Kehm et al., 2021]. Since AGEs are a very heterogenous group of compounds, individual AGEs, like pentosidine and Nε-(carboxymethyl)lysine can be measured using liquid and gas chromatography coupled to mass spectroscopy [Frijhoff et al., 2015].
Lipid oxidation products
Products of lipid oxidation have been widely used for the assessment of OS because polyunsaturated fatty acids (PUFAs) are very prone to oxidation in the presence of ROS due to their double bonds. The biochemical pathways of single PUFA oxidation can result in the formation of over 100 different volatile and semivolatile compounds, which can be classified into alkanes, alkenes, aldehydes and their derivatives, carboxylic acids, esters, furans and epoxides, as presented in a mechanistic study of Ratcliffe et al. [2020]. This study can open the possibility of finding new biomarkers of OS using the analysis of volatile organic compounds (VOCs).
Aldehydes originating from lipids exhibit high reactivity, leading them to readily engage with proteins to create Michael adducts, commonly referred to as advanced lipoxidation end products (ALEs) [Frijhoff et al., 2015]. To date, the most commonly analyzed lipid peroxidation products include malonaldehyde (MDA) and 4-hydroxynonenal (4-HNE). Both these markers can be measured using enzyme-linked immunosorbent assay (ELISA) kits as well as deploying the chromatographic methods [Ligor et al., 2015; Zhang et al., 2019]. MDA, alkenals, and alkadienals collectively form the group known as thiobarbituric acid-reactive substances (TBARS). These substances can undergo a reaction with two equivalents of thiobarbituric acid (TBA), resulting in the formation of a pink adduct complex. This complex can be conveniently measured using colorimetric or fluorometric assays [Marrocco et al., 2017]. However, due to its low specificity, this method received a lot of criticism [Frijhoff et al., 2015].
The interaction of PUFAs in membrane phospholipids with free ROS results in the formation of F2-isoprostanes (F2-IsoPs), which are chemically stable prostaglandin-like isomers. Initially generated within lipid membranes as a consequence of OS, F2-IsoPs are subsequently released in free form through phospholipase action. Notably, their measurement is not influenced by dietary lipid content [Marrocco et al., 2017]. These molecules serve as dependable markers for evaluating in vivo OS status [Dreiβigacker et al., 2010; Il’yasova et al., 2004]. Analyzing F2-IsoPs in biological fluids and exhaled breath condensate provides an estimation of total body production, while examining F2-IsoPs esterified in specific tissues offers insights into the localization and quantification of OS in those areas. It is crucial to consider that measurements of both MDA and 15(S)-8-iso-prostaglandin F2α by gas chromatography-mass spectrometry in plasma samples may be notably compromised in the presence of hemolysis [Dreiβigacker et al., 2010]. As mentioned before, F2-IsoPs can be measured using chromatographic methods as well as commercially available immunoenzymatic tests.
Nucleic acid modification products
ROS and RNS are the main sources of induced DNA damages. This process results in various DNA modifications, including nucleotide oxidation, strand breakage, loss of bases, and the formation of adducts [Dizdaroglu et al., 2002]. The hydroxyl radical (HO•) is capable of reacting with all purine and pyrimidine bases, as well as the deoxyribose backbone, yielding diverse products, the major of which being 7,8-dihydroxy-8-oxo-2′-deoxyguanosine (8-oxo-dG) [Marrocco et al., 2017]. As for RNA damage, the resulting 7,8-dihydro-8-oxo-guanosine is considered a marker of OS, associated with diabetes and neurodegenerative diseases [Broedbaek et al., 2011; Jorgensen et al., 2013]. The markers of nucleic acid oxidation can be measured both in bloodstream and in urine using chromatographic and immunoenzymatic methods [Marrocco et al., 2017].
Markers of reactive oxygen species generation
Certain enzymes that produce ROS, usually found intracellularly, can also be detected in the bloodstream, regardless of the mechanism behind their release. Among these enzymes, xanthine oxidase (XO), myeloperoxidase (MPO) and NADPH oxidases (NOX) stand out (Figure 2). Elevated circulating levels of ROS-generating enzymes may potentially lead to an increased production of ROS. However, this outcome depends on various factors, including the availability of the substrate and whether the ROS generated by these enzymes surpass the antioxidant defense mechanisms [Frijhoff et al., 2015]. In some instances, the formation of a metabolite or a reaction product is applied to measure the enzyme activity in vivo.
Figure 2
The simplified chemical reactions catalyzed by the reactive oxygen species (ROS)-producing enzymes. XO, xanthine oxidase; MPO, myeloperoxidase; NOX, NADPH oxidases.
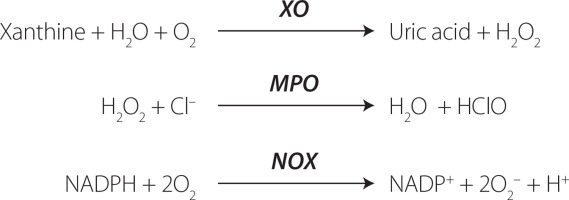
XO plays a role in the oxidation of xanthine to uric acid, producing ROS, specifically H2O2 [Nishino et al., 2008], rather than previously thought superoxide anion (O2•─) [Frijhoff et al., 2015]. The recognition of XO involvement in ischemia-reperfusion injury has led to clinical trials with XO inhibitors in cardiovascular disease, prompting investigations into measuring circulating XO [Yapca et al., 2013]. It is worth noting that XO inhibition not only impacts ROS production but also has additional effects, such as reducing hyperuricemia by decreasing uric acid levels, which could potentially improve cardiovascular diseases. Uric acid, while acting as an antioxidant, also exhibits proinflammatory properties through NALP3 inflammasome activation [Martinon et al., 2006]. During OS, XO is converted from xanthine dehydrogenase (XDH), which then produces ROS. In physiological conditions, there is a thermodynamic equilibrium between XDH and XO [Nishino et al., 2008]. XDH can transform into XO through the thiol oxidation, and the formation of a disulfide bond or proteolysis can then lock the enzyme in the XO form [Nishino et al., 2008].
Another ROS-inducing enzyme is myeloperoxidase (MPO), which is a peroxide enzyme containing heme pigment, mainly expressed in neutrophils, and is the only human enzyme capable of producing hypochlorous acid (HOCl). The MPO/HOCl system plays a crucial role in the neutrophil defense system against the microorganisms [Aratani, 2018]. HOCl is generated from H2O2 and induces the chlorinative stress due to its high oxidative activity, reacting with lipids, proteins and DNA [Chen et al., 2020]. Elevated levels of MPO are implicated in the development of various cardiovascular diseases [Aratani, 2018; Marrocco et al., 2017]. To use MPO as a marker, the level of specific products of MPO/HOCl system should be measured, such as 3-chlorotyrosine [Afshinnia et al., 2017].
NADPH oxidase (NOX) is a group of membrane-bound enzymes. Seven distinct NOX isoforms have been identified in humans (NOX1, NOX2, NOX3, NOX4, NOX5, DUOX1, and DUOX2) [Magnani & Mattevi, 2019]. Alongside the mitochondrial enzymes of the respiratory chain, NOXs are acknowledged as the primary contributors to cellular ROS generation. They generate superoxide (NOX1-3, NOX5) or hydrogen peroxide (NOX4, DUOX1-2) through an NADPH-dependent mechanism [Magnani & Mattevi, 2019]. Deficiency in NOX may result in immunosuppression, absence of otoconogenesis, or hypothyroidism. Conversely, elevated NOX activity is implicated in numerous pathologies, notably cardiovascular diseases and neurodegeneration [Bedard & Krause, 2007]. The measurement of NOX activity has many limitations; however, some attempts have been made to develop more reliable assays using, i.a., electron paramagnetic resonance, as described by Zielonka et al. [2017].
Markers of antioxidant defense system
Antioxidant enzymes
Antioxidant enzymes play an important role in protecting cells from the damaging effects of ROS and OS, thereby maintaining redox homeostasis. These enzymes neutralize ROS and prevent oxidative damage to cellular components, including lipids, proteins, and nucleic acids. The main antioxidant enzymes comprise SOD, CAT, and glutathione-dependent enzymes such as glutathione peroxidase (GPx), glutathione reductase (GR) and glutathione transferase (GST) [Marrocco et al., 2017].
SODs constitute a family of enzymes catalyzing the dismutation of O2 into oxygen and hydrogen peroxide, subsequently processed by other enzymes like catalases. Regarded as the most potent antioxidant enzyme, SOD exhibits a high catalytic rate and high resistance to physico-chemical stress [Bafana et al., 2011]. SODs can be categorized into four distinct groups based on the metal cofactors found in their active sites: copper-zinc-SOD (Cu,Zn-SOD), iron SOD (Fe-SOD), manganese SOD (Mn-SOD), and nickel SOD [Bafana et al., 2011]. These various forms of SODs can be found in different organisms across biological kingdoms and reside in different subcellular compartments. The metallic cofactor plays a crucial role in SOD activity, as depicted in Figure 3. SOD activity can be evaluated by analyzing the inhibition of the reduction rate of a tetrazolium salt through the O2 generated by a xanthine/XO enzymatic system, pyrogallol autoxidation, and the commercially available ELISA assays [Karim et al., 2018; Marrocco et al., 2017].
Figure 3
The simplified chemical reactions catalyzed by the antioxidant enzymes. SOD, superoxide dismutase; CAT, catalase; GR, glutathione reductase; GPx, glutathione peroxidase; GST, glutathione transferase; GSH, reduced glutathione; GSSG, oxidized glutathione; GSR, conjugate of GSH with electrophiles; R, electrophile.
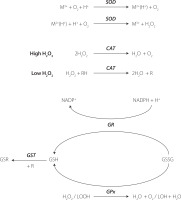
CAT is an enzyme that converts hydrogen peroxide into water and oxygen, playing a crucial role in reducing hydrogen peroxide generated by SOD and other metabolic processes in peroxisomes, and that exhibits different effects based on hydrogen peroxide concentrations [Sepasi Tehrani & MoosaviMovahedi, 2018]. At high levels, CAT converts two molecules of hydrogen peroxide in a single reaction due to its catalytic activity. At lower concentrations, it demonstrates peroxidatic activity, oxidizing hydrogen donors, such as alcohols, heavy metals, and hormones (Figure 3). Its activity can be measured using spectrophotometric assays [Bártíková et al., 2017; Karim et al., 2018; Marrocco et al., 2017; Papierska et al., 2022].
Glutathione serves as the primary redox agent in the majority of aerobic organisms, existing in reduced (GSH) and oxidized (glutathione disulfide, GSSG) states. The ratio between GSH and GSSG is commonly used to measure the cellular OS using commercial assays and high-performance liquid chromatography [Chatuphonprasert et al., 2019]. GPx oxidizes GSH to GSSG during reduction of H2O2 or organic hydroperoxides to water and alcohols. Subsequently, GSSG is reduced to GSH via reaction of GR, utilizing the reducing power of NADPH [Deponte, 2013]. Functioning as an intracellular antioxidant, glutathione interacts with electrophiles (R) forming GSR compounds through the enzymatic action of GST. A simplified pathway of glutathione metabolism is presented in Figure 3. The activity of glutathione-dependent enzymes can be measured using the fixed-time assay measuring H2O2 consumption and the continuous monitoring of GSSG formation [Marrocco et al., 2017].
Nonenzymatic antioxidant capacity
Apart from enzymes, there are various nonenzymatic compounds possessing antioxidant capacity, including endogenous substances (uric acid, thiols, bilirubin) and exogenous compounds derived from the diet, such as polyphenols, ascorbic acid, tocopherols and others [Marrocco et al., 2017]. Various methods can be deployed to measure antioxidant capacity, expressed as moles of oxidants neutralized per litre of body fluid. Detailed information about assays used to measure nonenzymatic antioxidant capacity (NEAC), also known as total antioxidant capacity (TAC), can be found elsewhere [Ialongo, 2017; Pellegrini et al., 2020]. Therefore, only selected, commonly used methods will be described below. Two types of methods can be distinguished based on the possible reactions: (1) hydrogen atom transfer (HAT), where the quenching capacity of free radicals by hydrogen donation is measured, and (2) single electron transfer (SET), where the reducing capacity toward any molecule by electron donation is measured. The chemical reaction and examples of each method are presented in Figure 4.
Figure 4
Classification of the methods used for the measurement of total antioxidant capacity (TAC) [Ialongo, 2017; Pegg et al., 2007].
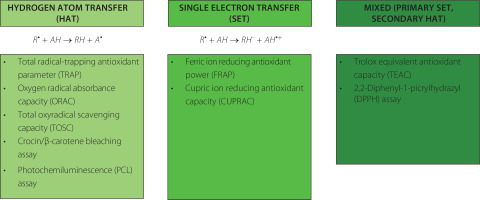
Oxygen radical absorbance capacity (ORAC) is a method that measures the oxidative degradation of a fluorescent molecule (either β-phycoerythrin or fluorescein) in a mixture containing free radical generators, such as azo-initiator compounds. The ORAC assay can only measure substances capable of trapping peroxyl-radicals [Fraga et al., 2014]. It has been commonly used to analyze TAC in biological fluids [Człapka-Matyasik & Ast, 2014], and to measure the antioxidant potential of food products [Chitisankul et al., 2022].
Another assay is the total radical-trapping antioxidant parameter (TRAP), conducted in aqueous solutions using 2,2′-azobis(2-methylpropionamidine) dihydrochloride (AAPH) as a thermolabile stoichiometric and water-soluble azoradical generator. This method has been successfully applied to measure the antioxidant capacity in the plasma collected from patients with atherosclerosis and hepatitis [Niculescu et al., 2001; Ozenirler et al., 2011].
Cupric ion reducing antioxidant capacity (CUPRAC) was proposed in 2004 as a method developed to measure the antioxidant capacity of vitamin C and E, and polyphenols [Apak et al., 2004]. The method involves mixing the analyzed sample with a copper(II) chloride solution, a neocuproine alcoholic solution, and an ammonium acetate aqueous buffer at pH 7, followed by measuring absorbance at 450 nm after 30 min. This method has been applied to measure TAC in serum samples [Apak et al., 2010] and is commonly used for the measurement of TAC in food products [Karadag et al., 2020].
The photochemiluminescence (PCL) assay involves the synergistic combination of photochemically generated free radicals with sensitive detection through chemiluminescence [Pegg et al., 2007]. It relies on the photo-induced autoxidation inhibition of luminol by antioxidants, mediated by the radical anion superoxide. This method is suitable for assessing the radical scavenging properties of individual antioxidants and more complex systems in the nanomolar range. Luminol serves as both a photosensitizer and an oxygen radical detection reagent. The PCL assay is performed separately for antioxidant capacity in water (ACW) and in lipids (ACL). ACW represents the TAC of water-soluble compounds, such as flavonoids and vitamin C, while ACL measures the activity of lipid-soluble compounds, including tocopherols and carotenoids. The PCL assay has been used to measure the TAC in biological fluids after strawberry consumption in animal study [Żary-Sikorska et al., 2021] and to determine the TAC of fortified gluten-free products [Drabińska et al., 2018; Krupa-Kozak et al., 2021].
EFFECT OF KETOGENIC DIET ON THE OXIDATIVE STRESS
Mitochondria play a key role in determining cellular energy production through oxidative phosphorylation. In this process, the electron transport chain activity contributes to the synthesis of cellular adenosine triphosphate (ATP). Electrons traverse these complexes, establishing a transmembrane proton gradient linked to ATP synthesis through ATP synthase. In many advanced malignancies, there is an upregulation of glycolysis and glucose uptake, with a notable diversion of the glycolytic end product (pyruvate) away from the mitochondrial tricarboxylic acid cycle. Instead of entering the cycle, pyruvate is preferentially reduced to form lactate, concurrently generating nicotinamide adenine dinucleotide (NAD+). This metabolic adaptation allows glycolysis to persist even in the presence of normal oxygen levels (normoxia). Contrary, KD has been found to reduce the production of ROS by suppressing glucolysis [Milder & Patel, 2012]. For example, cancer cells, unlike healthy cells, exhibit elevated glucose metabolism and alterations in mitochondrial oxidative metabolism, believed to result from persistent metabolic OS [Alhamzah et al., 2023]. It is theorized that the increased level of ROS is the primary reason for mitochondrial susceptibility to mutations. Ketone bodies are exclusively metabolized in the mitochondria; therefore, cells with damaged mitochondria, like cancer cells, are unable to utilize them to produce energy [Poff et al., 2013]. Succinyl-CoA: 3-ketoacid CoA-transferase (SCOT) is not produced by cancer cells, preventing ketone body metabolism and potentially leading to cancer cell death [Sawai et al., 2004]. Consequently, the inhibitory effect of KD on OS has spurred special interest in auxiliary cancer therapies [Alhamzah et al., 2023]. This section presents a summary of findings obtained through in vitro, in vivo and clinical trials aimed to assess the effect of KD on OS and mitochondrial functions.
In vitro studies
In countering OS, β-hydroxybutyrate serves as a natural inhibitor of class I and class IIa histone deacetylases (HDACs) [Shimazu et al., 2013]. To confirm that, human embryonic kidney 293 (HEK293) cells were incubated with varying levels of β-hydroxybutyrate for 8 h. The findings from this study revealed that inhibiting HDACs facilitates the transcription of detoxifying genes, including CAT, mitochondrial SOD, and metallothionein, providing protection against OS. Moreover, HDAC inhibition was associated with comprehensive alterations in transcription, involving genes responsible for oxidative stress resistance factors, such as FOXO3A and MT2. The exposure of cells to β-hydroxybutyrate led to enhanced histone acetylation at the promoters of forkhead box O3 (Foxo3a) and metallothionein-2 (Mt2), and the activation of both genes occurred with the selective depletion of HDAC1 and HDAC2. In line with heightened FOXO3A and MT2 activity, mice treated with β-hydroxybutyrate exhibited significant protection against OS [Shimazu et al., 2013].
Another cell line, i.e., HT-22 cells of the hippocampus, was exposed to varying doses of β-hydroxybutyric acid to investigate its neurotropic effects, OS markers, mitochondrial function, and apoptosis [Majrashi et al., 2021]. At a moderate dose (250 µM), β-hydroxybutyric acid exhibited a substantial increase in the viability of hippocampal neurons. Moreover, β-hydroxybutyric acid demonstrated antioxidant effects by reducing prooxidant markers of OS, including ROS and nitrite content, while elevating glutathione content, resulting in decreased lipid peroxidation. Additionally, the study revealed that β-hydroxybutyric acid enhanced mitochondrial functions by increasing the activities of Complex-I and Complex-IV. Moreover, it significantly reduced caspase-1 and caspase-3 activities, indicating a potential protective effect against apoptosis [Majrashi et al., 2021].
In a study with cortical primary cultures obtained from Wistar rat embryos treated with a physiological and a non-physiological isomer of β-hydroxybutyrate, both isomers demonstrated the ability to reduce ROS production. However, only the physiological ketone body stimulated ATP production [Julio-Amilpas et al., 2015]. Interestingly, in a dose-dependent evaluation, 10 mmol/L of β-hydroxybutyrate was found to elicit the highest neuroprotective effects. Another ketone body, i.e., acetoacetate, was addressed in a study of Board et al. [2017]. The authors compared the utility of acetoacetate and glucose by mesenchymal stem cells and found that acetoacetate was oxidized 35 times faster than glucose. Moreover, the production of ROS during oxidation was 45 times lower in the case of acetoacetate than glucose.
The molecular mechanism behind glucose deficiency-induced cytotoxicity and the protective role of β-hydroxybutyrate using SH-SY5Y cells was reported by Lamichhane et al. [2017]. Cell viability significantly decreased under glucose deficiency, accompanied by elevated ROS production and reduced phosphorylation of extracellular signal-regulated kinase (ERK) and glycogen synthase 3 (GSK3β). Inhibition of ROS reversed glucose deficiency-induced cytotoxicity and restored the diminished phosphorylation of ERK and GSK3β. β-Hydroxybutyrate reversed cytotoxicity, ROS production, and the decrease in phosphorylation of ERK and GSK3β induced by glucose deficiency. The authors have concluded that glucose deficiency-induced cytotoxicity involves ERK inhibition through ROS production and that this process is attenuated by β-hydroxybutyrate [Lamichhane et al., 2017].
The impact of the VLCKD on ROS production and cell viability was assessed in vitro by exposing Hep-G2 cells to sera obtained from patients with obesity following VLCKD for 8 weeks [Valenzano et al., 2019]. Significant effects on body weight, adiposity, and blood chemistry parameters were observed in the participants due to the dietary intervention, with a noticeable reduction in visceral adipose tissue. The viability of Hep-G2 cells remained unaffected after 24, 48, and 72 h of incubation with patients’ sera, both before and after the VLCKD. Additionally, ROS production was not significantly affected by the dietary treatment, which demonstrated that the short-term mild dietary ketosis did not exhibit cytotoxic effects and had no effect on OS [Valenzano et al., 2019].
In vivo studies
A KD has been found to elevate hippocampal glutathione biosynthesis in rats, enhancing mitochondrial antioxidant capacity [Jarrett et al., 2008]. This effect has been linked to the activation of NF E2-related factor 2 (Nrf2), a transcription factor that responds to OS by upregulating genes associated with antioxidant pathways, including those related to GSH synthesis and conjugation. Following a 3-week diet, rats on the KD exhibited Nrf2 nuclear accumulation and enhanced activity of its target NAD(P)H: quinone oxidoreductase (NQO1), in the hippocampus [Milder et al., 2010].
In a mouse study, mitochondrial uncoupling protein (UCP) activity was measured to check whether KD can affect the ROS production through affecting UCP [Sullivan et al., 2004]. The maximum rates of mitochondrial respiration were markedly higher in animals administered a KD compared to those on a standard diet, suggesting an augmentation of UCP-mediated proton conductance capable of reducing ROS production. Western blots revealed significant or nearly significant increases in protein levels of UCP2, UCP4, and UCP5, with elevated immunoreactivity to these three UCP isoforms, particularly observed in the dentate gyrus of KD-fed mice. Furthermore, they observed a significant reduction in oligomycin-induced ROS production in KD-fed mice, which was not observed in the control group. The authors concluded that the mechanism of reduction of ROS production is associated with the activation of mitochondrial UCPs [Sullivan et al., 2004].
The combination of KD with radiation was found to reduce tumour growth in mice with lung cancer [Allen et al., 2013]. Notably, tumors of animals fed with KD exhibited elevated oxidative damage, predominantly through lipid peroxidation, confirmed by the presence of 4HNE-modified proteins. Additionally, there was a reduction in proliferation, as indicated by decreased immunoreactive proliferating cell nuclear antigen. It suggests that KD may enhance the efficacy of radiotherapy in cancer treatment [Allen et al., 2013].
Significant increases in hippocampal NAD+/NADH ratio and blood ketone bodies were detected in rats fed with KD after 2 days and remained elevated at three weeks, indicating an early and persistent metabolic shift [Elamin et al., 2017]. According to the authors, an increased NAD during ketolytic metabolism may be a primary mechanism of potential health-beneficial effects of KD.
The study by Julio-Amilpas et al. [2015] investigated the effect of β-hydroxybutyrate on the neuronal death induced by severe non-coma hypoglycemia in rats. A reduction in ROS production was noted in distinct cortical areas and subregions of the hippocampus, which resulted in the prevention of neuronal death in the cortex. The authors have concluded that the protective effects of ketone bodies stem not only from its metabolic actions but also from its ability to diminish ROS, positioning β-hydroxybutyrate as a promising candidate for addressing ischemic and traumatic injuries.
Another study analyzed the effect of KD on the OS in rat with traumatic brain injury [Greco et al., 2016]. Following injury, OS significantly increased at 6 and 24 h, and this effect was mitigated by the KD. The KD also induced the expression of antioxidant proteins, including NQO1 and SOD. The authors have concluded that KD enhance cerebral metabolism post-traumatic brain injury by providing alternative substrates and exerting antioxidant effects, thereby preventing OS-mediated mitochondrial dysfunction [Greco et al., 2016]. A study with a spinal cord injury showed similar effects [Wang et al., 2017]. Two-week intervention with KD or other-day fasting resulted in the 31–43% inhibition of the spinal cord HDAC activity and the enhanced expressions of acetylated histone AcH3K9 and AcH3K14 and genes associated with anti-oxidative stress, such as Foxo3a and Mt2. Consequently, the content of SOD, Foxo3a and CAT proteins increased. Notably, a reduction in the MDA concentration was noted, which was suggested to contribute to the neuroprotection of the spinal cord against oxidative damage [Wang et al., 2017]. The positive effect of KD on the elevated OS caused by injury was also observed in a rat model of carotid artery balloon-injury [Xu et al., 2023]. The KD suppressed OS caused by the injury. This was evident in the decreased levels of ROS, MDA, and MPO activity, with simultaneous increase in the SOD activity [Xu et al., 2023].
A very comprehensive study, analyzing the short- and long-term effects of KD on the multi-organ OS and mitochondria functions in rats, was reported by Kephart et al. [2017]. Both KD and the standard diet supplemented with exogenous ketone bodies were followed for one week and eight months. The rats on a short-term KD diet had significantly higher levels of ketones in their blood, indicating that the diet itself (not ketone bodies supplementation) caused an increase in ketones. In short-term, the rats fed with KD and exogenous ketone bodies had higher liver antioxidant capacity. In the long term, the KD-fed rats showed higher liver antioxidant capacity and higher expression of GPx compared to standard diet, as well as the lowest level of liver protein carbonyls. Contrary, KD negatively affected skeletal muscle mitochondrial physiology with higher production of ROS in the mitochondria of the gastrocnemius muscle [Kephart et al., 2017].
A recent study evaluated the effect of KD on OS in young rats with diet-induced obesity [Drabińska et al., 2022]. In this study, rats were fed with a calorie-restrictive KD or a standard diet for four weeks. At the end of experiment, there was no difference in AGEs and MDA, while an increase in SOD activity was recorded in the KD-fed rats. The observed changes were inconclusive and do not allow to definitely claim about KD effect on OS [Drabińska et al., 2022]. No effect on the OS was reported in another study with rats following KD or a standard diet throughout the lifespan [Parry et al., 2018]. The length of life was longer in the rats fed with KD compared to the control animals (762 vs. 624 days), and the activity of citrate synthase, representing estimated volume of mitochondria, was higher in the KD-fed rats. However, no differences were detected in various OS markers, including SOD, CAT, GPx, 4-HNE and carbonyls [Parry et al., 2018].
The composition of low-carbohydrate diets was evaluated in a study by Kaburagi et al. [2019]. Diets consisting of lard vs. MCT oil were compared in non-obese mice fed ad libitum for 13 weeks. Increased renal weight, glomerular hypertrophy, and enlargement of intraglomerular small vessels with wall thickening were observed in both groups. In the kidney, the level of Nε-(carboxymethyl)lysine was significantly lower in the mice fed a lard-based diet. Contrary, the concentration of Nε-(carboxyethyl) lysine was the lowest in the MCT-fed mice [Kaburagi et al., 2019]. This study underlined the importance of diet composition, not only the ratio between fat and other nutrients.
A testicular OS was a focus of interest in a recent study conducted by Üstündağ et al. [2023]. These authors evaluated the effects of KD and intermittent fasting, separately and together, on testicular health. In the KD-fed rats, an increase in body and testis weight was observed, along with elevated testosterone and reduced oestradiol levels. KD alone as well as combined with intermittent fasting exhibited positive effects on OS by lowering MDA and MPO levels, while increasing glutathione, CAT, and nitric oxide in testicular tissue, suggesting that KD may have a positive effect on the male reproductive system [Üstündağ et al., 2023].
Clinical trials
The number of clinical trials evaluating the effect of KD on OS is much lower than of in vitro and in vivo investigations. In a study with healthy women, KD was applied for 14 days to evaluate their blood redox status [Nazarewicz et al., 2007]. At the end of the study, although participants had the body mass index (BMI) within a normal range, weight loss was observed; however, without the differences in the fat mass. Moreover, an increase in TAC, uric acid, and sulfhydryl content was observed, while the activity of SOD and CAT as well as MDA content remained unmodified. The authors have concluded that even a short-term intervention with KD increased the TAC without increasing OS. It is particularly interesting, since the adaptation of ketosis has not been achieved in this study and it is surprising that so vivid changes were observed in healthy subjects.
Another study was performed in a group of 18 Taekwondo players following KD for three weeks [Rhyu et al., 2014]. There was no notable difference observed in body composition, ROS, and SOD levels between the individuals following KD and the control group. However, the KD group exhibited an increased high-density lipoprotein (HDL) cholesterol level, while the control group demonstrated elevated lactate dehydrogenase and MDA levels after three weeks. The authors have implied that weight loss due to three weeks of calorie restriction and exercise may induce OS and that the KD could be beneficial in preventing it [Rhyu et al., 2014]. However, it has to be kept in mind that the adaptation to ketosis takes at least 4 weeks; hence, it was also not achieved in this study.
KD is applied mainly as a therapy for the drug-resistant epilepsy. A recent study evaluated the effect of three-month KD on the OS and the seizure incidence in 40 pediatric patients with epilepsy [Poorshiri et al., 2023]. Out of 40, 34 children completed the study, and among them, 21 had a 50% reduction in seizure frequency after KD. The serum levels of OS parameters, including MDA and 8-oxo-dG, were significantly reduced after KD implementation. The KD appears to decrease brain OS by elevating ketosis, which was negatively correlated with OS markers. The authors have suggested that the reduction of OS can be responsible for the potential anti-seizure mechanism of KD [Poorshiri et al., 2023].
The analysis of the clinical trial database (clinicaltrial.gov) indicated that there are two ongoing studies evaluating the effect of KD on OS and mitochondrial functions in subjects with obesity. In the first study, the study protocol of which can be found in my previous work [Drabińska et al., 2023], the OS markers, including MDA, uric acid, SOD, F2-IsoPs and 8-oxo-dG, will be analyzed in samples collected from 80 women following KD for 8 weeks. The second study will measure mitochondrial functions and OS in 63 participants with obesity divided into four groups: (1) KD group; (2) calorie-restricted standard diet group; (3) intermittent fasting 16/8; and (4) usual diet [U.S. National Institutes of Health, 2023]. The intervention will be followed for one month. Taking into account that the intervention part had been completed in both studies, we can expect the results in near future.
SUMMARY AND FUTURE PERSPECTIVES
The presented review discussed the significance of redox homeostasis and its intricate metabolic pathways. Despite the growth in knowledge over the last decades, OS remains not fully understood. Numerous studies, conducted in vitro and on animal models, have shown the potential of the KD in alleviating the adverse effects of OS, particularly in situations where OS is elevated, such as injuries. However, the clinical effects of KD and its impact on redox homeostasis are not yet fully elucidated. More long-term studies, conducted with larger groups and sufficient sample sizes, are needed. The adaptation to ketosis, which can last from 4 to 6 weeks, should be considered when planning such studies. Appropriate adaptation to ketosis may yield different results than those observed in short-term dietary interventions. Moreover, considering the multidimensionality of redox balance, studies focused on OS should analyze multiple markers simultaneously to observe all the by-products resulting from ROS-mediated modifications. This includes also measuring the activity of enzymes involved in ROS generation and antioxidant defense, as well as assessing the antioxidant potential of the organism itself. As the metabolic effects of KD are not fully understood, it is worthwhile to analyze different enzymes and by-products generated to determine which group of compounds is most susceptible to KD interventions. Finally, both the short-term and long-term effects of KD should be evaluated, especially in cases where KD is applied for weight reduction, as it is often followed for only a short period but it may result in the consequences in future.