INTRODUCTION
Chicken meat is loved food by people all over the world due to its delicate taste and a high nutritional value. It contains essential nutrients, especially proteins with a well-balanced amino acid composition, free amino acids, peptides, and essential trace elements [Ali et al., 2019]. Chicken can be cooked in many ways, but chicken soup is usually an important part of an everyday diet of the Chinese people. While the chicken is cooked, some water-soluble components such as proteins, carbohydrates, vitamins, minerals, oligopeptides, and amino acids will be dissolved into chicken soup and their content in soup will increase with the prolongation of heating time [Meng et al., 2022; Zhang et al., 2021]. These compounds are dietary nutrients, but some of them also have biological activity. Small molecules of chicken soup, such as carnosine, anserine and taurine, can cause central nervous system excitement, improve antioxidant and immune capacity, increase appetite, and promote digestion [Xiao et al., 2021]. After cooking chicken soup for a long time, native structures of nutrients, such as proteins and carbohydrates, are destroyed and become more easily digestible and absorbable by the human body.
The flavour and texture are important factors affecting consumers’ acceptance and preference for soups. The flavour in chicken soup is developed by the thermal reaction of carbohydrates, proteins and lipids in the cooking process, mainly including the Maillard reaction, lipid oxidation, and the interaction between the reaction products [Qi et al., 2023; Zhang et al., 2018]. During this process, flavour precursors, such as water-soluble components and lipids, are dissolved, released, and interact to form volatile and non-volatile compounds [Dashdorj et al., 2015]. Research has shown that stewing conditions, such as time, heating temperature, and heating rate, affect a soup’s flavour profile during cooking. Therefore, in recent years, several researches published have focused on the influences of cooking conditions on the flavour components and nutrients in soups [Pérez-Palacios et al., 2017; Zou et al., 2021]. Qi et al. [2018] studied the effect of stewing time on taste activity and volatile compound levels in chicken soup. The results have shown that increasing cooking time reduces the content of taste components but increases the aroma level. Our previous study also found that after cooking for 4 h, the changes of flavour components in chicken soups tended to stabilise [Guan et al., 2023]. Zhang et al. [2013] investigated the influence of stewing temperature on protein hydrolysates and sensory properties of crucian carp soup and found that the soup made at 85°C had excellent sensory qualities in colour, flavour, and nutritional value. Rotola-Pukkila et al. [2015] reported that the heating temperature had a more significant influence on the extraction and release of umami compounds in pork soup than the cooking time. In addition to flavour, texture of the soup is also an essential factor of its quality assessment.
However, the literature available has limited information on the rheological properties and flavour compound formation in soups obtained by different “Huohou” modes. “Huohou” is the most critical technical term in traditional Chinese cooking, originally meaning temperature and duration of heating required for dishes from raw to cooked, and is a key factor affecting cooking results. Chotechuang et al. [2018] reported that different combinations of boiling temperature and time can affect chicken bone stock quality. This work aimed to evaluate the influences of different “Huohou” modes on the rheological properties and formation of flavour compounds of Dagu chicken soup. Analyses of the zeta potential, particle size, viscosity, 5′-nucleotide content, and the profiles of amino acids, fatty acids, and volatile compounds in four samples, enabled determining the effect of stewing modes on the physicochemical quality and flavour compound formation of the chicken soup. In this study, based on the traditional experience of making soup in China and our pre-experimental findings, we used four different “Huohou” modes commonly used to stew Chinese Dagu chicken soup, namely, the high fire short time (HFST, 100°C, 1 h), the medium-high fire mid-length time (MFMT, 98°C, 2 h), the medium fire long time (MFLT, 90°C, 3 h), and the low fire ultra-length time (LFUT, 83°C, 4 h).
MATERIALS AND METHODS
Materials and chemicals
The freshly slaughtered Chinese Dagu chickens were provided by Dagu Chicken Breeding Farm Co., Ltd. (Zhuanghe, China). The chicken carcasses were placed in polyethene bags and transported to the laboratory on ice. Reference standards of amino acids, inosine 5′-monophosphate (5′-IMP), adenosine 5′-monophosphate (5′-AMP), guanosine 5′-monophosphate (5′-GMP), n-alkanes (C7 to C30), and cyclohexanone were obtained from Sigma-Aldrich Co., Ltd. (Shanghai, China). Additionally, sulphuric acid, 4-dimethylaminobenzaldehyde, chloramine T, chloroform, methanol, and sodium chloride were purchased from China National Medicines Co., Ltd. (Beijing, China). All other chemicals used were of analytical grade.
Sample preparation
Each half of the chicken carcass (approximately 800 g) was cut into evenly sized pieces that were put into a high-temperature cooking bag (nylon/polypropylene, moisture-proof, resistant to high temperature, and shock-resistant). Next, clearwater twice the weight of the chicken was added, and the bags were sealed under vacuum [Qi et al., 2017]. The sealed samples were divided into four groups based on different “Huohou” modes, each was placed in an electrical stewpot with different powers, and the same volume of water was added to the pot. The first group was prepared using the stewing model of high fire short time (HFST): heated from room temperature (approximately 23°C) to boiling temperature (100±0.15°C) by an electrical stewpot with a constant power of 1,300 W, then kept at the constant temperature for 1 h. The second group was prepared using the stewing model of medium-high fire mid-length time (MFMT): heated from room temperature to 98±0.15°C by an electrical stewpot with a constant power of 800 W, then kept at the constant temperature for 2 h. The third group was prepared using the stewing model of medium fire long time (MFLT): heated from room temperature to 90±0.16°C by an electrical stewpot with a constant power of 500 W, then kept at the constant temperature for 3 h. The fourth group was prepared using the model of low fire ultra-length time (LFUT): heated from room temperature to microboiling temperature (83±0.16°C) by an electrical stewpot with a constant power of 300 W, then kept at the constant temperature for 4 h. After stewing, the liquid was filtered to remove chicken solids, and the chicken soup was ready.
Total solid and collagen content determination
The content of total solids was determined according to Guan et al. [2023]. The solid content was expressed as g/100 mL of soup. The collagen content of the samples was measured as reported by Barido & Lee [2021]. The sample (4 g) was hydrolysed with 30 mL of 3 M sulphuric acid and diluted to a final volume of 250 mL with distilled water. The 4 mL of hydrolysate solution was mixed with 2 mL of 4-dimethylaminobenzaldehyde solution (10%, w/v,) and 2 mL of chloramine T solution (7%, w/v,) in a test tube. The mixtures were heated at 60°C for 20 min, and then cooled at room temperature for 20 min. The absorbance was measured at 558 nm using a spectrophotometer (UV-2550, Shimadzu Co., Kyoto, Japan). The collagen content was calculated by multiplying the hydroxyproline content and 11.1 (the coefficient of hydrolysis of collagen to hydroxyproline), and the result was expressed as mg/mL soup. The content of hydroxyproline was determined according to the standard curve.
Zeta potential and particle size estimation
The zeta potential and particle size of the four samples were evaluated using a Zetasizer Nano ZS-90 analyzer (Malvern Instruments Ltd., Malvern, UK). The volume-weighted mean diameters (d4,3) and surface-weighted mean diameters (d3,2) were recorded.
Rheological behaviour measurements
Rheological measurements were carried out according to the method previously used by Zhu et al. [2020]. A rotary rheometer (Discovery HR-1, TA Instruments, New Castle, DE, USA) was used to measure the static and dynamic rheological properties. To this end, 1 mL of the chicken soup was placed on the parallel plate under the rheometer, and the upper parallel plate with a diameter of 40 mm was lowered slowly. The distance between the two parallel plates was 1 mm, and the excess liquid flowing out of the plate was erased. The sample was balanced for 2 min before measurements. Static rheology was used to record the variation of viscosity of the soup with a shear rate (0.1–1000 1/s) at 25°C and test interval of 1,000 μm. The dynamic rheology was measured in the linear viscoelastic region, and the oscillating stress was fixed. The relationship between shear rate, viscosity, and shear stress was analysed. The correlation between the shear rate and shear stress was determined using Equation (1):
where: τ (Pa) is shear stress, γ (1/s) is shear rate, K (Pa×s) is consistency coefficient, and n represents flow index.Free amino acid analysis
Free amino acids (FAAs) were determined according to the method described by Qi et al. [2017] with some modifications. Chicken soup (2 mL) was mixed with 3% (w/v) sulfosalicylic acid (4 mL), and the mixture was centrifuged at 4°C for 15 min (14,000×g). Then, 2 mL of n-hexane was mixed with the supernatant and left to stand for 15 min and 2 mL of the aqueous phase was filtered using a 0.22 μm membrane. The FAAs were analysed in filtrate using an automatic amino acid analyzer (L-8900, Hitachi, Tokyo, Japan). The post-column derivatization was carried out with ninhydrin at 135°C. Each FAA was identified by comparing its retention time with a standard amino acid and quantified using an external standard method. The content of FAAs was expressed as mg/100 mL soup. Taste activity values (TAVs) were calculated as the ratio of the content of an individual amino acid in the chicken soup to its taste threshold value obtained from the reported literature [Meng et al., 2022].
5′-Nucleotide analysis
5′-Nucleotides were determined as described by Qi et al. [2021] using a high-performance liquid chromatography (HPLC) system (E2695, Waters Ltd., Milford, CT, USA) equipped with an X Bridge C18 (5 μm, 4.6×250 mm). Chicken soup (10 mL) was mixed with perchloric acid (30 mL, 5% w/v), then the mixture was centrifuged at 4°C for 15 min (10,000×g). The supernatant was adjusted to pH 4.5 with 1 M sodium hydroxide solution, diluted to 100 mL, and filtered through a 0.22 μm membrane prior to the HPLC analysis. The chromatographic separation was carried out using eluent A (0.05 M KH2PO3 pH 5.4) and eluent B (methanol) in a gradient system (98% A for 14 min, 85% A for 7 min, and 98% A for 9 min) with a flow rate of 1.0 mL/min. UV detector wavelength was 254 nm. The 5′-GMP, 5′-IMP, and 5′-AMP were quantified by comparing the peak area of the nucleotide with that of the external standard, and the results were expressed as g/100 mL of the soup. TAVs were calculated as the ratio of the content of an individual nucleotide in the chicken soup to its taste threshold value obtained from the reported literature [Qi et al., 2017].
Determination of fatty acid composition
The total lipids were extracted using the method of Folch et al. [1957]. Chicken soup (20 mL) was mixed with 400 mL of a chloroform-methanol solution (2:1, v/v), and then filtered after vortexing for 10 s. A saturated sodium chloride solution was mixed with the filtrate and placed for 3 h at 4°C. The chloroform from the lower phase was removed through a rotary evaporator at 45°C, whereas the remaining material was the total lipids.
According to the procedure previously described by Diao et al. [2017], with some modification, the lipids were converted to fatty acid methyl esters. Briefly, 50 mg of lipids were dissolved with n-hexane (2 mL) and a sodium methoxide regent (2 M, 0.4 mL) was mixed, then saturated sodium chloride (3 mL) was added. After shaking for 15 s, the mixture was left for 10 min, and the supernatant (1 μL) filtrated through a 0.22 μm filter membrane was injected into the 7890-5975 gas chromatography-mass spectrometry (GC-MS) system (Agilent Co., Ltd., Santa Clara, CA, USA) equipped with an Agilent INNOWAX capillary column (30 m × 0.32 mm × 0.25 μm, Thermo Fisher, Waltham, MA, USA). The injection temperature and detector temperature were 250°C and 230°C, respectively. Helium was used as a carrier gas with a flow rate of 1 mL/min. Oven temperature program was as follows: 140°C (2 min) − 200°C (6°C/min, 2 min) − 230°C (2°C/min, 2 min) − 250°C (4°C/min, 2 min). The fatty acids were matched with the National Institute of Standards and Technology (NIST) 147 library spectra, and those with similarity greater than 90% were selected as identification results. Peak area normalisation method was used for quantitative analysis [Petenuci et al., 2019], and the relative content of individual fatty acids was expressed as g per 100 g of total fatty acids.
Volatile compound analysis
The volatile compounds in chicken soups were determined as described by Guan et al. [2023] by means of the head space-solid phase microextraction-gas chromatography-mass spectrometry (HP-SPME-GC-MS) method. To this end, 5 g of the chicken soup and 2 μL of cyclohexanone (1.11 μg/μL) were put into a headspace bottle and SPME fibre (75 μm CAR/PDMS, Supelco, Bellefonte, PA, USA) was used to collect the flavour compounds from different samples at 50°C for 30 min. Subsequently, the fibre was inserted into the GC injector port and desorbed at 250°C for 5 min. The GC oven temperature program was as follows: initial temperature 40°C (3 min) − 70°C (3°C/min) −180°C (5°C/min) − 280°C (10°C/min, 5min). The carrier gas – helium – was used with a constant flow rate of 1 mL/min. The retention time of each compound was converted into a linear retention index (LRI) using n-alkanes as a reference. The volatile compounds were identified by comparing LRI values with those reported in the literature [Qi et al., 2017] and the data listed in authentic online databases [http://www.flavornet.org, http://www.odour.org.uk]. The quantification of each volatile compound was carried out by comparing its peak area with that of the internal standard (cyclohexanone), and the content was expressed ng/g of the soup.
The odour activity values (OAVs) of volatile compounds were expressed as the ratio between the concentration of a volatile compound and its threshold value reported in the aqueous phase [Qi et al., 2017]. A compound with an OAV greater than or equal to 1 was considered to be an aroma contributor [Bi et al., 2021].
Analysis using electronic tongue and electronic nose
The taste of the samples was measured using an electronic tongue (SA402B e-tongue, Insent, Atsugi, Japan), which had six lipid membrane sensors. To enable the analysis, 35 mL of the chicken soup sample from each group were transferred into a special sample cup and then placed on the automatic sampler of the electronic tongue according to the set sequence. The single sampling time was 120 s, once per second. Each group of samples was repeatedly tested 4 times. The taste characteristics of the last three times of data collection were analysed. The response values of the e-tongue were recorded and analysed using principal component analysis (PCA).
The volatile compounds in the chicken soup were analysed by the electronic nose (PEN3 e-nose, Airsense Analytics, Schwerin, Germany) equipped with ten different gas sensors (Table 1) [Zhang J.X. et al., 2022]. The e-nose sensor was pre-heated and calibrated before the test. After a stable sensor response signal, the sample (10 mL) was placed into a precision-threaded vial (20 mL). After 30 min-enrichment at room temperature, the volatile chemicals in the sample bottle reached saturation. The test was repeated five times, and the last three response values were used as valid data. The response values of the e-nose were analysed using PCA.
Table 1
Performance of the sensor arrays of the e-nose.
Sensory evaluation
Chicken soups were tested for sensory characteristics by 10 panelists (5 women and 5 men, aged between 22 and 25) from the Bohai University, Jinzhou, China. The panelists with sensory evaluation experience were recruited and trained in recognizing and describing the intensity ratings of standard references, and three sensory attributes (umami, bitterness and aroma) were used for sensory evaluation. Based on the reported literature [Liang et al., 2022; Qi et al., 2021] with some modifications, the reference materials were 0.15% monosodium glutamate (umami), 0.08% quinine (bitterness), and 100 g of Chinese Dagu chicken leg meat stewed in 200 g of water at 98°C for 2 h (aroma). The training sessions were carried out 4 times for 2 h each time before the sensory analysis. The samples were maintained between 55 and 60°C in a water bath during testing and were randomly named. The panelists assessed the umami, bitterness and aroma of each sample, based on 6-point intensity scales (1–2, weaker; 2–3, weak; 3–4, middle; 4–5, strong; and 5–6, stronger). Panelists rinsed their mouths with drinking water (50 mL) between assessments to eliminate the effects of fatigue and carryover. All panelists scored separately without interference with each other, and the average value was taken as the result.
Statistical analysis
All data was presented as the means ± standard deviations (SD) of three independent replicates. The results were analysed by one-way analysis of variance using SPSS 19.0 (IBM, Armonk, NY, USA), followed by Duncan’s multiple range test with a significance level of p<0.05. The data from the e-nose and e-tongue were analysed through Origin Pro 2021 software (OriginLab Corporation, Northampton, MA, USA).
RESULTS AND DISCUSSION
Solid content and collagen content
The solid content in broths is one of the main indexes used to assess their quality, and it also reflects the overall dissolution effect of nutrients (such as protein, fat, amino acid, etc.) during the cooking process of raw materials. The effects of different stewing modes on the solid content of chicken soup are shown in Table 2. The solid content of the chicken soup prepared under different stewing modes was significantly different, and the solid content of the chicken soup stewed by MFLT (90°C, 3 h) was the highest. This may be due to the fact that the solid substance released in the soup mainly come from soluble matter, such as collagen, minerals, glycogen, vitamins, etc., which can rapidly dissolve from chicken’s tissues with the extension of stewing time at the higher temperature. Subsequently, insoluble large molecules could be released from the deeper tissues, as was shown in a previous study during tuna head soup preparation [Qian et al., 2019]. However, the chicken soup stewing by LFUT took a long time, and the solid content was lower. This is most likely due to the slow dissolution rate of solids in the meat under the lower stewing temperature. Rotola-Pukkila et al. [2015] noted that the cooking temperature played a more significant role than the cooking time in analysing the content of umami compounds in pork meat juice.
Table 2
Solid content, collagen content, particle size, and zeta potential of the chicken soups prepared with different stewing modes.
[i] Data are expressed as mean ± standard deviation (n=3). Different lowercase letters (a–d) in the same column indicate significant differences (p<0.05). HFST, high fire short time; MFMT, medium-high fire mid-length time; MFLT, medium fire long time; LFUT, low fire ultra-length time; d3,2, surface-weighted mean diameter; d4,3, volume-weighted mean diameter.
Collagen in a denatured state is easy to agglutinate into a gel, thus affecting the viscosity of the soup. Collagen in the hot dissolved state will also make the soup more mellow. The content and dissolution of collagen in chicken soup are related to the stewing temperature and time. Different stewing modes had a significant effect on the collagen content of the chicken soup (Table 2). The highest (p<0.05) collagen content, reaching 5.60 mg/mL, was determined in the chicken soup obtained using the MFLT technology. It might be because medium fire stewing for 3 h was more conducive to collagen dissolution.
Particle size and zeta potential
The d4,3 and d3,2 of particles of each chicken soup prepared under different stewing modes are presented in Table 2. The results showed that, compared with HFST, MFLT, and LFUT soups, the d4,3 and d3,2 of the particles of the soup prepared using the MFMT technology were smaller. It indicated that the MFMT technology could promote the migration of more components from meat to the soup and the formation of a stable emulsion under the effect of continuous high temperature processing, thus decreasing the particle size [Guan et al., 2023]. Therefore, it was inferred that the stewing modes and dissolving ingredients had strong influences on the particle size in the chicken soup. Also, Diao et al. [2016] reported that the increase of d4,3 and d3,2 was due to the formation of larger droplet-coalesced aggregates by those individual droplets, which greatly reduced the emulsification effect.
The zeta potential of four kinds of soups is depicted in Table 2. The absolute value of zeta potential of the soup stewed by MFMT was the highest (8.66 mV). A high zeta potential (absolute value) reflects higher stability of the soup. The zeta potential affects the repulsive force between particles. Qiu et al. [2015] demonstrated that the low zeta potential of emulsion droplets decreased electrostatic repulsion between neighbouring protein-coated droplets, leading to droplet polymerization. Moriyama et al. [2003] also showed that the larger the zeta potential, the smaller particles in the emulsion. In this research, the high zeta potential of chicken soup stewed by MFMT contributed to the small particle sizes.
Viscosity
Qi et al. [2023] noted that the viscosity was an important indicator of emulsion stability. Furthermore, Zhang M. et al. [2022] pointed the increase of viscosity contributes to the physical stability of emulsions, which may be due to the lower mobility reducing the chance of particle collision. As noted in Figure 1A, with the increase in shear rate, the viscosity of chicken soup rapidly decreased and gradually stabilized, indicating that the chicken soup had the property of shear-thinning and pseudoelasticity. With the increase in shear rate, the water environment around the substances in soups was destroyed, and the interaction force was weakened, which changed the viscosity of the chicken soup and caused shear thinning. At the same shear rate, the viscosity of the chicken soup stewed by MFMT was significantly higher than that of the other soups. This may be because the chicken soup obtained by MFMT technology had a small particle size. Similar results have been noted by Costa et al. [2019], who demonstrated that the smaller the particle size in yogurt, the better its dispersion and the higher its viscosity value. Coutinho et al. [2019] also reported that the particle size greatly influenced the viscosity of chicken soup. Figure 1B shows that the shear stress of four chicken soups prepared under different stewing modes increased with the increase in shear rate. The non-linear relationship between shear stress and shear rate indicated that the four soups were non-Newtonian fluids. Furthermore, the yield stress occurred in the four samples, and the shear stress of the soup stewed by HFST was lower compared to the other soups. One possible explanation was that the interaction of particles in the chicken soup obtained by the HFST technology formed a weak network structure, and small yield stress was required to destroy the structure [Huang et al., 2020].
Free amino acid composition
FAAs, as indispensable precursor substances for producing meat flavour, play an important role in the modulation of chicken soup palatability. It has been reported that the enhancement in the FAAs of chicken soup may be due to the enhanced migration of FAAs from boiled meat into the soup [Qi et al., 2017]. The delicious taste of soups is not determined by a single kind of amino acids. The balance and interaction between different FAAs, such as sweet amino acids, umami amino acids and bitter amino acids, are the key factors determining the taste of soups. The FAAs composition of the chicken soups prepared with different stewing modes is shown in Table 3. The 17 FAAs identified in the soups were classified into four groups on the basis of their taste (umami, sweet, bitter, or tasteless) [Meng et al., 2022]. Compared with HFST, MFMT, and MFLT, the total FAAs content in the soup stewed by LFUT was the highest, and the content of bitter amino acids was higher than that of umami amino acids, sweet amino acids and tasteless amino acids. The number of bitter amino acids increased significantly due to the increased hydrolysis of proteins and peptides in the crucian carp as the heating temperature increased [Zhang et al., 2013]. Meng et al. [2022] also noted that shortening heating time appropriately was necessary to reduce the formation of bitter amino acids and maintain a good taste of bone soup. However, the taste active values (TAV) of the bitter taste FAAs (valine, methionine, isoleucine, leucine, phenylalanine, lysine, and arginine) were low, less than 1 (Table 3); hence, they had little impact on the taste of the chicken soups.
Table 3
Free amino acid (FAA) composition and their taste activity values (TAVs) of the chicken soups prepared with different stewing modes.
[i] Data are expressed as mean ± standard deviation (n=3). Different lowercase letters (a-d) in the same row (separately for content and TAV) indicate significant differences (p<0.05). “–”, no reference threshold. HFST, high fire short time; MFMT, medium-high fire mid-length time; MFLT, medium fire long time; LFUT, low fire ultra-length time.
Umami substances can balance food’s taste and overall flavour. The high content of umami compounds can make food more palatable. According to the TAV values of FAAs (Table 3), glutamic acid was the main contributor to the umami taste of the chicken soups. Wu & Shiau [2002] also reported that glutamic acid was the predominant free amino acid in chicken soups produced from chicken meat. Zhuang et al. [2016] also found that glutamic acid, glycine, and alanine were the major flavour amino acids in cooked crab meat. In our study, the content and TAV value of glutamic acid in the chicken soup from the MFMT group was higher than that of the soups from the other three groups (LFUT, MFLT, and HFST). The lower umami taste FAAs in LFUT and MFLT groups might be explained by Maillard’s reaction because of long-time stewing. While the reason for the low content of umami taste FAAs in the HFST group may be because the short time was not enough to enable the migration of all original FAAs from chicken meat into the soup.
5′-Nucleotide content
5′-Nucleotide has a significant impact on the flavour of meat products and has been widely used as a food flavour enhancer. The nucleotides in chicken soup come from the thermal transfer of nucleotides of chicken meat, which contributes to the chicken soup’s flavour [Qi et al., 2022]. The synergies between nucleotides also play a major role in improving overall flavour. The contents of 5′-IMP, 5′-GMP, and 5′-AMP were quantified in the chicken soups prepared with different stewing models (Table 4). 5′-IMP was the most important umami nucleotide in the four kinds of chicken soup because its TAV was greater than 1. Additionally, as reported by Kawai et al. [2002], 5′-IMP interacts with a large number of sweet amino acids, such as serine, glycine, and alanine, which have a strong enhancing effect on the umami taste. As shown in Table 4, the 5′-IMP content of the soups of the MFMT group was the highest, while the soups of the HFST group had the lowest 5′-IMP content. This may be due to the fact that rapid heating rate and high temperature of the HFST stewing mode led to the accelerated degradation of 5′-IMP to convert inosine and hypoxanthine during cooking. Zou et al. [2018b] also reported that increasing heating temperature promoted the soup’s 5′-IMP degradation. Furthermore, Zou et al. [2018a] have reported that the synergy of 5′-GMP and 5′-IMP enhanced the umami taste of food. Consistent with 5′-IMP, the content of 5′-GMP in the chicken soups of the MFMT group was higher than in the other groups. The contents of 5′-AMP in the soups of MFMT and LFUT groups were higher than that in the other two groups, but there was no significant difference between these two groups (p>0.05). Although the TAVs of 5′-AMP and 5′-GMP in the four soups were less than 1, the synergistic effect of 5′-AMP and 5′-GMP on umami induction should be considered.
Table 4
5′-Nucleotide contents and their taste activity values (TAVs) of the chicken soups prepared with different stewing modes.
[i] Data are expressed as mean ± standard deviation (n=3). Different lowercase letters (a-d) in the same row (separately for content and TAV) indicate significant differences (p<0.05). HFST, high fire short time; MFMT, medium-high fire mid-length time; MFLT, medium fire long time; LFUT, low fire ultra-length time; 5′-GMP, guanosine 5′-monophosphate; 5′-IMP, inosine 5′-monophosphate; 5′-AMP, adenosine 5′-monophosphate. Taste thresholds were obtained from literature [Qi et al., 2017].
Fatty acid composition
Fatty acids are significant precursors of flavour components that affect soup’s flavour. Various individual fatty acids have been reported to have important flavour characteristics [He et al., 2023]. Table 5 shows data indicating the influence of different stewing modes on the content of fatty acids in chicken soup. A total of 23 fatty acids were detected in the chicken soups, including 10 saturated fatty acids (SFA), 6 monounsaturated fatty acids (MUFA), and 7 polyunsaturated fatty acids (PUFA). Among them, the main SFA were palmitoleic acid (C16:0) and stearic acid (C18:0), and the main unsaturated fatty acids (USFA) were oleic acid (C18:1) and linoleic acid (C18:2). Han & Zhang [2019] showed that SFA could mask bitterness, whereas Cameron et al. [2000] showed that pork flavour correlated positively with the MUFA content. As shown in Table 5, compared to the other stewing modes (HFST, MFLT, and LFUT), the sum of SFA in the chicken soup prepared in the MFMT stewing mode was higher. The relative contents of PUFA in the four samples from high to low were LFUT, MFLT, MFMT, and HFST. This result may be due to the fact that the high-temperature stewing (HFST) accelerated the lipid oxidation rate, which changed the unsaturated fatty acid carbon chain and converted them into aldehydes and alcohols, such as 2-heptanal, 2-nonenal, and 2-octanal [Kim et al., 2020].
Table 5
Compositions of fatty acids in the chicken soups prepared with different stewing modes (g/100 g total fatty acids).
[i] Different lowercase letters (a–d) in the same row indicate significant differences (p<0.05). All data are presented as the mean ± standard deviation. SFA, saturated fatty acids; MUFA, monounsaturated fatty acids; PUFA, polyunsaturated fatty acids; HFST, high fire short time; MFMT, medium-high fire mid-length time; MFLT, medium fire long time; LFUT, low fire ultralength time.
Volatile compound composition
The results of GC-MS analysis of volatile compounds in chicken soups with different stewing models are shown in Table 6. In total, 34, 36, 51 and 47 volatile compounds were identified in the four chicken soups prepared with stewing models of HFST, MFMT, MFLT, and LFUT, respectively, which indicated that new volatile compounds were formed along with stewing time extension. The volatile compounds from different samples mainly included aldehydes, hydrocarbons, esters, and alcohols. Ba et al. [2013] reported that aldehydes were produced upon the thermal oxidation and decomposition of unsaturated fatty acids. For example, hexanal and heptanal are produced by the oxidation of n-6 PUFA, and octanal and nonanal are produced by the oxidation of n-9 PUFA [Tanimoto et al., 2015]. As displayed in Table 6, aldehydes played an important role in the overall aroma of the cooked chicken soups because of their lower odour thresholds and higher contents. Among all aldehydes identified in the four soups obtained under the different stewing models, the hexanal content was the highest. Qi et al. [2017] also found similar results for Chinese yellowfeather chicken soup. Additionally, Yang et al. [2011] also noted that the hexanal content represented the flavour formation in cooked meat. Compared to other stewing modes, the chicken soup obtained under the mode of LFUT had the highest hexanal content. This may be because prolonged stewing (4 h) caused more lipids to dissolve from the chicken carcass into the soup and be oxidized to aldehydes.
Table 6
Volatile compound profile and odour activity values (OAVs) of chicken soups prepared with different stewing modes.
[i] Data are expressed as mean ± standard deviation (n=3). Different lowercase letters (a-d) in the same row (separately for content and OAV) indicate significant differences (p<0.05). HFST, high fire short time; MFMT, medium-high fire mid-length time; MFLT, medium fire long time; LFUT, low fire ultra-length time; LRI: linear retention index; NA, not acquired; ND, not detected. Odor thresholds were obtained from literature [Meng et al., 2022] and http://www.odour.org.uk.
Volatile compound composition
Saturated alkanes can be produced by the decarboxylation and cleavage of carbon-carbon bonds in higher fatty acids [Watanabe & Sato, 1971]. Although there were many kinds of alkanes in each chicken soup (Table 6), they did not contribute directly to the flavour due to their long carbon chain. Still, they played a specific role in improving the overall flavour.
Alcohols mainly come from the reduction reaction of carbonyl compounds and oxidation reaction of lipids, and exhibit grass, mushroom and earthy odours [Wan et al. 2021]. However, some alcohols and carboxylic acids in meat products react to form esters through esterification. As shown in Table 6, the alcohol content of the chicken soups was affected by stewing temperature and duration. Wettasinghe et al. [2000] reported that 1-octen-3-ol was one of the key odour compounds in roasted chicken skin due to its low odour threshold, and that changes in its content can affect the overall flavour. 1-Octen-3-ol, which contributed to mushroom and roasted aroma in chicken soup [Zhang et al., 2018], was detected in all the samples, and its odour intensity was the highest in the MFLT group soups compared to the other samples (HFST, MFMT, and LFUT). One possible explanation was that more lipids were decomposed to form 1-octen-3-ol in the MFLT stewing mode.
Additionally, only three esters were detected at low contents in the four samples by GC-MS, and they may have little impact on odour perception because of their high odour thresholds. Therefore, these volatile compounds were neglected.
Flavour analysed by e-nose and e-tongue
The e-nose system is sensitive to volatile compounds of the sample within the measurable range, and small changes in their content will lead to differences in sensor response. PCA is a technique that reduces the dimensionality of large datasets by creating new uncorrelated variables [Chen et al., 2022]. The volatile compounds of the four soups obtained under different stewing modes were presented in the PCA spatial distribution map (Figure 2A). The first two principal components (PCs) explained a total of 89.9% variation (PC1=83.9%, PC2=6.0%), which indicated that the two principal components contributed to the main feature information of different samples. As shown in Figure 2A, the soups of MFMT and MFLT groups were close to each other, indicating that they had similar odour characteristics. Additionally, MFMT and MFLT were far away from the samples of LFUT and HFST, which indicated that the odour characteristics of MFMT and MFLT samples were significantly different from those of the LFUT and HFST samples.
Principal component analysis was used to determine further differences in odour characteristics between the samples by performing a load analysis on the e-nose response (Figure 2B). Based on the response intensity of ten sensors to a specific characteristic gas, the main characteristic gas in each soup was tentatively speculated. The length of the arrow represents the contribution of compounds to the overall odour profile of the sample [Li et al. 2022]. The highest contribution rate in PC1 was found for the HFST sample, followed by the LFUT and MFMT samples, and the highest contribution rate in PC2 was found for the MFLT sample. Additionally, MFMT and MFLT had similar distances from the origin, indicating that they had similar characteristics, which was consistent with the results presented in Figure 2A. As shown in Figure 2B, the sensor’s response to hydrogen (W6S), alkanes (W3S), and ammonia (W3C) was higher for the HFST sample; methane (W1S), nitrogen oxides (W5S), and aromatic compounds and sulphur organic compounds (W2W) were the main contributors in the MFLT sample; the W5C sensor responded more strongly to the LFUT and MFMT samples, indicating that they contained higher levels of short-chain alkane aromatic ingredients [Zhang J.X. et al., 2022].
Figure 2
Principal component analysis (PCA) plot (A) and loading plot (B) of the e-nose data and PCA plot (C) of the e-tongue data. HFST, high fire short time; MFMT, medium-high fire mid-length time; MFLT, medium fire long time; LFUT, low fire ultra-length time; W1C, W5S, W3C, W6S, W5C, W1S, W1W, W2S, W2W, and W3S, sensor names (see Table 1 for details).
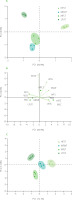
As shown in Figure 2C, the taste of the four chicken soup samples was discriminable, and there was no overlap in the 2D space. The total contribution variance of PC1 and PC2 was 85.1%, which meant that the first two PCs already contained sufficient information to reflect the total variance of the whole dataset. These data demonstrated that the e-nose and the e-tongue could discriminate the different samples. Different stewing modes significantly influenced the odour and taste of the chicken soups.
Sensory evaluation
The umami taste, bitterness and aroma of chicken soup have a significant impact on its flavour [Wu et al., 2023]. Therefore, the sensory evaluation was carried out to better understand the effects of different stewing models on the changes in taste and aroma of the soups (Table 7). It could be seen that the chicken soup stewed by MFMT received the highest score for umami taste, while the chicken soup obtained by MFLT and LFUT received the highest score for its aroma. Bitterness score of the soup stewed by MFLT compared to MFMT was also higher. Prolonging the stewing time appropriately helped to develop aroma, but at the same time, bitterness also increased. However, it was weak and did not affect the overall flavour of the chicken soup. The sensory evaluation results were consistent with the results of e-nose, the e-tongue, and GC-MS.
CONCLUSIONS
Different stewing modes (HFST, MFMT, MFLT, and LFUT) significantly affected chicken soup’s stability and flavour compound formation. The chicken soup obtained under the MFMT stewing mode had a high viscosity, small particle size, and higher zeta potential. The contents of the umami components (umami taste FAAs and nucleotides) in the MFMT samples were higher than in the other samples. However, the profile and contents of volatile compounds of the chicken soup prepared under the MFMT stewing mode were different and lower than in the LFUT and MFLT groups, indicating that low temperature and long-time stewing were beneficial to the formation of volatile compounds. Hence, when considering the stability and umami taste of chicken soup, and time-saving, it is appropriate to use the MFMT mode to prepare it.