INTRODUCTION
The recovery of food by-products is a strategy fully framed in the context of the circular economy, an alternative economic system to the linear model applied up to now, whose aim is to improve the efficiency of resources. This is particularly important in the case of animal production, given the high demand for natural resources and the generation of greenhouse gases. This impact on the environment is becoming a matter of great concern due to the increase in both the world population and the number of inhabitants with enough purchasing power to be able to include products of animal origin in their diet [FAO, 2022].
The edible internal organs obtained during the slaughter of animals are known as offal. Offal is present in many traditional regional cuisine dishes around the world, both for its nutritional value and for its organoleptic characteristics (flavour and texture). However, its consumption in Western countries has drastically declined in recent decades due to changes in consumer preferences. Increasing offal consumption has been suggested as a realistic strategy to reduce the contribution of meat production to environmental impacts, since fewer animals would need to be slaughtered. Therefore, the valorisation of non-meat edible parts obtained during the slaughter of animals with a lower value than meat but with good nutritional and/or techno-functional properties should cease to be an option and become an obligation due to the increase in demand for animal protein that is expected to happen soon [da Costa et al., 2019].
In the case of the pig, the offal yield is around 17% by live weight, among which the liver stands out; its nutritional value is equivalent to that of meat, but with a higher content of vitamins and minerals. This makes liver to be considered a first-grade meat by-product due to its richness in proteins, vitamins, and mineral elements [Kakimov et al., 2018]. However, a slight metallic taste together with a bitter taste which could be related to the presence of iron/blood and bile production, respectively, along with its less tender texture make pork liver less appetising. In addition, off-flavours can become intense during liver processing/ storage due to iron-catalysed oxidation of unsaturated fatty acids [Im et al., 2004].
Currently, proteins of plant origin are increasingly attractive compared to those of animal origin both because of the lower environmental impact in obtaining them and for ethical issues. However, we must not forget that the human species is essentially omnivorous. While reducing meat consumption at the individual level must be a reality to address the environmental challenges we face, large-scale meat production is unlikely to slow down in the face of projected population growth and taking into account the higher requirements of rich-protein foods that will come associated. For this reason, betting on an efficient use of the raw material must also be a priority in the meat industry. The great mismatch between the daily production of pork livers in slaughterhouses and the low demand from consumers forces us to seek alternatives for their use and recovery. An interesting way of valorising pork liver could be through obtaining protein fractions to use them as food ingredients, not only as meat extenders but also as techno-functional constituents. This work aims to deepen some characteristics of the livers supplied by an industrial slaughterhouse and, in particular, of their protein fraction, in order to obtain useful information for further studies focused on obtaining ingredients for food formulation. Therefore, the microbiological quality and the colour of livers, the solubility and the thermal properties of proteins, as well as the chemical composition of liver homogenates were determined in this study.
MATERIALS AND METHODS
Material handling
Every sampling day (six days in total), three fresh pork livers from healthy adult animals (Large White × Landrace × Pietrain × Duroc commercial crossbred; live weight of approximately 100 kg and 6 months old), regardless of sex, were supplied by a local abattoir (NORFRISA SA, Riudellots de la Selva, Spain), once their gallbladder was separated. At the slaughterhouse, each liver was placed separately in a sterile bag. Livers were transported and kept under refrigerated conditions for approximately half an hour, until they were processed upon arrival at the laboratory. First, each liver was visually inspected and weighed under sterile conditions (precision of 0.1 g). Next, 10 g of liver were placed in a sterile stomacher bag by taking a sample from each of the lobes in order to determine the microbiological contamination as described below. After that, pH was measured in quadruplicate using a Crison GLP 22 pH-meter (Hach Langue SLU, Ames, IA, USA) coupled to an insert-type electrode (2-Pore F, XS Instruments, Capri, Italy). The colour of some livers was measured as indicated below. Finally, the remaining blood vessels and the most evident connective tissue were completely removed from all livers.
Determination of total mesophilic aerobic bacterial counts
Counts of total mesophilic aerobic bacteria (MAB) were determined on 10 g-samples from each liver (n=18) weighed into a sterile stomacher bag under sterile conditions in a Telstar BV-100 laminar flow chamber (Telstar, Terrassa, Spain). Next, 90 mL of 10 g/L tryptone water (L42, Oxoid Ltd, Basingstoke, UK) with 5 g/L NaCl were added. After that, each sample was serially diluted in sterile tryptone water and plated on plate count agar (PCA, Oxoid Ltd) by the pour-plate method. The plates were then incubated at 30°C for 72 h. The results were expressed as log colony-forming unit (cfu) per g.
Instrumental colour analysis
Surface and inner colour of six different fresh livers was measured in quadruplicate in the CIELab colour space using a Minolta CR-400 colorimeter (Minolta Co., Ltd, Osaka, Japan) with an optical geometry d:0° (diffuse illumination/0° viewing angle) and equipped with a CIE D65 illuminant and a CIE 2° standard observer. The L* coordinate corresponded to lightness, while hue angle (h°) and chroma (C*) were calculated from the chromaticity coordinates a* [(–)greenness/(+)redness] and b* [(–)blueness/(+) yellowness] from Equations (1) and (2), respectively.
Determination of thermal properties by differential scanning calorimetry (DSC)
Samples from six different livers were individually analysed to determine their thermal properties using a Q2000 V24.4 Build 116 differential scanning calorimeter (TA Instruments, New Castle, DE, USA). Samples were accurately weighed (15–20 mg) into Tzero aluminium pans (40 μL), using a precision balance (±0.01 mg, Analytical Plus, Mettler-Toledo S.A.E., Cornellà de Llobregat, Spain), and sealed hermetically. Then, they were heated from 30°C to 100°C at a scan rate of 3°C/min. An empty aluminium Tzero pan served as a reference. The transition temperatures (°C) and the transition enthalpy (ΔH, J/g) were determined from the thermogram obtained using the Universal Analytics Program, version 4.7 (TA Instruments).
Protein solubility determination
Samples coming from three different livers were used to determine the protein solubility in the pH range from 4.5 to 8.5, taking measurements at each pH unit. For each liver, pieces of the different lobes were taken and ground with a household electric mixer to obtain a homogeneous sample. Then, 10% (w/v) protein dispersions were prepared at the required pH using different solutions: citric acid-Na2HPO4 (pH 4.5); KH2PO4-Na2HPO4 (pH 5.5–7.5); and Tris-HCl (pH 8.5). The dispersions were first homogenised using a Polytron PT 30 laboratory homogeniser (Kinematica AG, Malters, Switzerland) at 7,000 rpm for 3 min, then shaken for 30 min at 3,000 rpm at room temperature and finally centrifuged at 2,500×g for 15 min in a SORVALL RC 5C Plus centrifuge (Dupont, Newtown, MA, USA). Soluble protein content in the supernatant was determined by the Kjeldahl method [ISO, 1978]. Protein solubility was presented as g of soluble protein per kg of the total protein content of pork liver. Both soluble protein and total protein were determined in duplicate.
Physicochemical characterisation of pork liver homogenates
On each sampling day, after removing the connective tissue, each liver was cut into small pieces and a pork liver homogenate (PLH) was prepared by mixing and grinding equal amounts of the three livers (500 g final weight) at 2,100 rpm for 1 min with a Cutter Sammic CKE-5 food processor-emulsifier (Sammic S.L., Azkoitia, Spain). Moisture, fat, crude protein, and ash contents of all liver homogenates were determined according to the methods recommended by the American Oil Chemists’ Society [AOCS, 2009]. A specific factor of 6.25 was applied to convert total Kjeldahl nitrogen to crude protein content according to ISO 937:1978 procedure [ISO, 1978]. Elemental analysis was performed after acid hydrolysis of the ash using a SpectrAA Varian 50B atomic emission/absorption spectrophotometer (Varian Inc., Palo Alto, CA, USA) at a specific wavelength for each mineral (Na, 589.0 nm; K, 766.5 nm; Ca, 422.7 nm; Mg, 285.2 nm; Fe, 248.3 nm; Zn, 213.9 nm; Cu, 324.7 nm; and Mn, 279.5 nm). Non-protein nitrogen (NPN) and collagen contents were also analysed in liver homogenates from three of the sampling days. NPN was determined by Kjeldahl method as the fraction soluble in 12.5% (w/v) trichloroacetic acid (TCA). Total collagen was calculated by multiplying the hydroxyproline (Hyp) content by 8. Hyp content was determined using the Nordic Committee on Food Analysis–Association of Official Agricultural Chemists (NMKL– AOAC) colorimetric method described by Kolar [1990].
Statistical analysis
Mean and standard deviation (SD) were calculated for all the parameters analysed as statistical descriptors. For solubility data, sampling days were considered as blocks in a randomised complete block design in which pH was the main factor. This statistical analysis was carried out using IBM SPSS Statistics 28 (IBM Corporation International, Armonk, NY, USA). Data were submitted to the analysis of variance (ANOVA) using the general linear model procedure (PROC GLM). A polynomial contrast was applied when a significant effect was obtained. The significance level applied was α=0.05.
RESULTS AND DISCUSSION
Characterisation of fresh pork liver
The morphology of all livers used in this study completely matches that described in the scientific literature, without any anomalies. They were multilobar, with four main lobes (2 lateral and 2 medial, one right and the other left in each case) and adopting a typical trefoil shape. The caudate lobe, which is smaller and attached to the right lateral lobe was also observed in the visceral face of all livers. Some authors have described a sixth lobe known as the square lobe only visible on the visceral face; it is the smallest one [Ntonas et al., 2020]. In the present study, only the main lobes have been considered.
Pork livers weighed 1.72±0.18 kg as supplied by the industrial slaughterhouse, thus showing a variation coefficient ~10%. Liver weight depends on many factors including age and diet [Cliplef & McKay, 1993]; it also varies markedly among animals of similar weight due to differences in fasting time before slaughter or in the level of post-sacrifice bleeding of this organ [Loeffel & Koch, 1970]. In spite of that, our results are fully consistent with those found in the literature for healthy pigs intended for meat production, i.e. 6 months of age and weighing around 100 kg [Cliplef & McKay, 1993]. Accordingly, liver weight would represent ~1.7% of animal weight, a percentage that matches that reported for omnivores by Elefson et al. [2021]. At slaughter weight of pigs (100 kg), the liver is by far the heaviest organ of the red viscera group and being only exceeded by the small intestine when all viscera are considered together.
According to our results and as observed by other authors [Steen et al., 2016], the pH of pork liver was slightly acidic (6.38±0.16). Similar values have been obtained in the case of livers from other animal species also intended for human consumption such as cattle, buffaloes, and lambs [Devatkal et al., 2004]. However, some authors have also reported lower values for pork livers [Tomović et al., 2016]. Relatively low pH values are usually obtained in animals that have not been fasted or when measurements were not made shortly after the animal sacrifice.
Total mesophilic aerobic bacteria counts
The pork liver is thinner and smaller in volume compared to the liver of other animals, making its specific surface area relatively large, which can have implications from the point of view of their microbiological contamination. Mesophilic aerobic bacteria (MAB) are often tested for food safety. The livers analysed in this study showed MAB counts of 3.52±0.66 log cfu/g. In the European Union, there is no specific legislation for microbiological quality of offal. However, taking as reference the European Commission (EC) Regulation No 1441/2007 [European Commission, 2007] relative to the microbiological criteria applicable to food products and, specifically, for minced meat, the livers may be deemed suitable for human consumption. In spite of that, a rapid liver cooling and maintenance of the cold chain until their use are key aspects to minimise microbiological risks.
Colour
The colour of pork livers was low in lightness, with a predominance of the +a* component over the +b* component (Table 1). Our data also showed a significantly (p<0.05) yellower colour inside the livers relative to the surface, which translates into significant (p<0.05) changes in the value of h° and C* parameters, both higher at the inner region. The obtained values fully match the reddish-brown or light brown attribute that is frequently used to describe the colour of fresh and healthy pork livers. Conversely, the colour coordinates of pork liver are very different from those of duck liver, a product with a much higher commercial value, which is lighter, less red, more yellow and with a predominance of the +b* component over the +a* component [Fernandez et al., 2010].
Thermal properties
Knowing the transition temperatures and the enthalpies of phase transitions associated to liver proteins can be useful to determine the effects of different processes on their native conformation as well as the potential of using them as techno-functional ingredients due to the effects of heating on their rheological properties. In this study, the thermal properties of pork liver proteins were estimated from the DSC thermograms, which showed a complex thermal behaviour (Figure 1). At temperatures <40°C, an endothermic thermal transition was observed in all the thermograms with the minimum of the peak at 32.5±0.05°C (not shown), which could correspond to the fat melting [Sasaki et al., 2006]. At higher temperatures, two other endothermic peaks together with two shoulders (T1–T4) were also observed, which could be related to the protein denaturation. These thermal transitions occurred in the temperature range from 48°C to 96–97°C, with the most important one taking place around ~67°C. The specific temperature for each transition is indicated in Figure 1. The thermal transitions associated with the denaturation of rat liver proteins occur in a temperature range similar to that of pork liver; moreover, the range for liver samples was also wider than that obtained for muscle tissue samples [Ritchie et al., 1994].
Figure 1
Differential scanning calorimetry profile of pork liver samples from 30 to 100°C. ΔH, transition enthalpy; T0, onset temperature; T1–4, transition temperatures related to protein denaturation. Values are shown as mean and standard deviation (n=6).
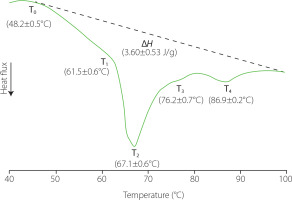
The liver is one of the most metabolically active organs, involved in many physiological functions. This makes its proteome very complex. Bovo et al. [2018] identified approximately 500 different proteins in pork liver. Blood is also found in liver with hepatic blood volume accounting for 10% to 15% of the total volume [Lautt, 1977]. This means that haemoglobin can be also present in a relatively high percentage. In addition, transitions linked to other macromolecules or supramolecular structures present in cells or liver tissue could also contribute to the thermal transitions observed in the DSC profile in this range of temperatures [Ritchie et al., 1994].
For all liver samples, the last endothermic transition reached a minimum peak value close to 90°C, which shows that some of the liver molecules were highly thermally stable. Haemoglobin in the liver samples could contribute to this thermal transition; the DSC thermograms of this protein present two endothermic peaks with a bigger one around 78–79°C and the other at 90°C. In addition, the deoxyribonucleic acid (DNA) unfolding could also contribute to this transition; normally, it is considered to take place around 85°C [Yan & Iwasaki, 2004]. In this sense, it should be considered that liver consists of several cell types, with hepatocytes constituting 70–80% of the total liver cell population and representing around 80% of the total liver volume [Racanelli & Rehermann, 2006; Vekemans & Braet, 2005].
Protein solubility as a function of pH
A high protein solubility is desirable both for the economic viability of the recovery process and for the use of the extracted proteins as techno-functional ingredients. In the present study, protein solubility was determined at pH values ranging from pH 4.5 to pH 8.5, i.e., a range that does not include overly aggressive conditions. As it was shown in Figure 2, protein solubility of about 400 g/kg was obtained at the lowest pH tested (pH 4.5), while at the highest pH (pH 8.5) approximately 900 g/kg of the proteins were solubilised. A high protein solubility at alkali conditions has been also observed for other offal [Gault & Lawrie, 1980]. In addition, the protein solubility increased successively along with pH increase, following a linear trend, as indicated by the results obtained by applying a polynomial contrast (p<0.05). An asymmetrical parabolic shape in the solubility profile of proteins from both animal and vegetal sources has been often reported, with the minimum solubility occurring near to the isoelectric point (pI) due to the prevalence of proteinprotein interactions [Hrynets et al., 2011; Khalid et al., 2003]. Isoelectric points in the range from 4.5 to 6.8 have been described for different pork liver proteins [Canduri et al., 1988; Yamada et al., 1981]. Therefore, the low solubility at pH 4.5 could be not surprising. In addition, although protein solubility increases both below and above the pI, normally the increase at pH values below the pI is more abrupt than at higher pHs. The successive increase observed in this study would be consistent with this behaviour. In addition, it would be in agreement with the results obtained by da Costa et al. [2019] also for pork liver proteins.
Figure 2
Solubility of pork liver proteins as affected by pH. Values are shown as mean and standard deviation (n=3).
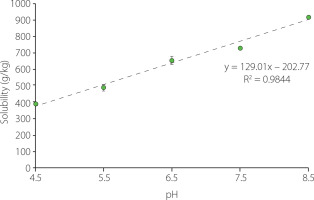
Acidic or negatively charged amino acids contribute more favourably to protein solubility than basic or positively charged, polar neutral and non-polar amino acids, in this order respectively [Trevino et al., 2007]. Seong et al. [2014] showed that basic and non-polar amino acids accounted for 17 and 42% of the total amino acids, respectively, while acidic and polar amino acids together represented 41% in pork liver. Because aspartic acid/ asparagine and glutamic acid/glutamine were non differentiated, it was not possible to quantify these last ones separately. According to Kramer et al. [2012], negative surface charge positively correlates with protein solubility, probably due to strong binding of water by the acidic amino acids. Average pKa values of 3.5±1.2 and 4.2±0.9 for Asp and Glu, respectively, have been reported by Grimsley et al. [2009]; the dispersion in these values is due to the effect of the local environment on the pKa of the ionisable groups in folded proteins. Therefore, they are mostly negatively charged in the tested pH range. However, pKa values of basic amino acids are found mostly outside the tested range (Lys, 10.4; Arg, >12); only in the case of histidine does it fall within the range (His, 6.6±1.0). This means that many of the basic groups will be positively charged at the tested pHs. The number of acidic and basic groups charged in the protein molecules will therefore be a determinant factor of their solubility.
Chemical composition of pork liver homogenates
The chemical composition of the pork liver homogenates (PLH) is shown in Table 2. Moisture, fat, protein, and ash contents accounted all together for ~98% of the total composition. The rest would correspond to carbohydrates. Although water is the most abundant component, its content was relatively low compared to most offal, including the small intestine, lung, spleen, stomach, and heart [Alfaia et al., 2020]. As expected, PLHs were rich in crude protein (180–190 g/kg). These results completely agree with those obtained by other authors [Saguer et al., 2019; Steen et al., 2016; Venegas Fornias, 1996], but higher (210–250 g/kg) and lower (~140 g/kg) contents have also been reported [Babicz et al., 2019; Nuckles et al., 1990; Seong et al., 2014]. Variation in liver protein content may be related to breed, age, and nutrition pattern. Importantly, the protein content of pork liver obtained in this study was very similar to that reported for fresh pork lean meat [Jiménez Torres et al., 2013]. Liver recovery is particularly attractive considering that it does not only contain all the essential amino acids, but also that they meet the requirements of the Food and Agriculture Organization (FAO) [FAO, 2013; Zou et al., 2018]. Essential amino acids in pork liver proteins are close to 50%, according to Venegas Fornias [1996]. Seong et al. [2014] found that both pork liver and pancreas proteins were richer in essential amino acids compared to other edible offal such as spleen and heart. In addition, liver is low in fat, with levels comparable to the lean parts of pork [Alfaia et al., 2020; Jiménez Torres et al., 2013; Saguer et al., 2019; USDA, 1992]. Phospholipids are the most abundant lipid class in pork liver; specifically, they represent more than 75% of total lipids, and phosphatidylcholine accounts for about 60% of total phospholipids [Hunter et al., 1973]. In fact, pork liver is one of the richest foods in phospholipids, surpassed only by egg yolk and beef brain [Zheng et al., 2018]. Phospholipids are the main components of biological membranes, but are also found in bile, exclusively as phosphatidylcholine in pork liver [Alvaro et al., 1986].
Table 2
Chemical composition of pork liver homogenates.
Carbohydrate content is usually calculated by difference. Saguer et al. [2019] estimated a content of ~15 g/kg, while a value of ~25 g/kg was indicated in the United States Department of Agriculture (USDA) report [USDA, 1992]. These differences may be partially due to the specific protocols applied to animals before slaughter and may have some implications from a food safety point of view; livers from slaughtered animals with lower glycogen content have a higher pH [Warriss & Bevis, 1987]. Liver cells store large deposits of glucose in the form of glycogen. However, glycogen is consumed during fasting time [Warriss & Bevis, 1987].
As measured, crude protein represents the nitrogen-containing fraction, so it can include NPN-containing molecules. A low non-protein nitrogen (NPN) content was obtained for PLH (Table 2), which represented 5.2±0.1% of the total nitrogen. In land animals, NPN compounds usually make up no more than 10%. However, their content could gradually increase during storage under refrigeration conditions due to endogenous and exogenous proteolytic enzyme activity [Custódio et al., 2016; Silva et al., 2020]. Pork liver is particularly rich in nucleic acid nitrogen and exhibits a high purine content [Kaneko et al., 2014]. This could be important because purines have effects on liver taste. It is well-accepted that high-purine foods are umami foods [Johnson et al., 2013]. This savoury taste enhances flavour properties and is also able of supressing bitterness and heightening saltiness. In addition, umami has also been shown to improve appetite but also satiety [Masic & Yeomans, 2014].
The collagen content of PLH is shown in Table 2. It was low, particularly if the content was expressed in relation to total protein content (3.7%). Babicz et al. [2019] reported a similar percentage, while Nuckles et al. [1990] determined even lower values (3.4 g/kg that represented 1.5% of total protein). This can be considered an important difference in relation to other viscera. Connective tissue in pork lungs, stomach, small and large intestines represents approximately 20% of total protein [Gault & Lawrie, 1980]. However, this tough connective tissue means that the consumption of pork liver is not as popular as that of other species (beef, calf, or chicken). In the pork liver – unlike the liver of other animals intended for human consumption – the connective tissue is visible because it is surrounding its lobules, forming a fibrous envelope that interconnects the adjacent portal areas. In addition, it can also be found within lobules, both adjacent to the sinusoids and around the central veins [Mik et al., 2018].
The liver, together with the pancreas and spleen, are the pork offal with the highest ash content [Alfaia et al., 2020]. In the present work, an average content of 13 g/kg has been determined (Table 2), which agrees with those most frequently cited in the literature [Alfaia et al., 2020; Seong et al., 2014], although Steen et al. [2016] recorded a slightly higher content (19.7 g/kg). Its contribution to the nutritional richness associated with liver consumption is due to the abundance of some essential trace elements. It is widely accepted that mineral elements tend to accumulate in some offal such as liver and kidney. Liver is a rich source of iron, zinc, copper, and manganese [Babicz et al., 2019; Mulvihill, 2014; Stasiak et al., 2017; Tomović et al., 2019]. In addition, it is very rich in some macroelements, such as calcium, sodium, and potassium. As it was shown in Table 2, the liver content of iron far exceeds zinc. With some exceptions [Stasiak et al., 2017], this agrees with what is mostly reported in the literature [Alfaia et al., 2020; Tomović et al., 2011]. Opposite is true for meat [Tomović et al., 2011]. Both iron and zinc in meat and meat products are considered highly bioavailable while their absorption from plant foods is quite limited, probably due to the presence of phytates that act as antinutritional agents blocking mineral absorption [Mulvihill, 2014]. Iron deficiency is the most common nutritional disorder in the world, in both developed and developing countries [Mulvihill, 2014]. Globally, anemia prevalence was 22.8% in 2019, but being the highest among children under five years [Gardner & Kassebaum, 2020]; adolescent girls and pregnant women are also at high risk of deficiency. The actual tend to shift to a plant-based diet from the animal protein diet could have some health implications.
Iron and zinc are the two most abundant trace minerals in the human body. Iron plays a key role as part of haemoproteins, but it is also present in some important proteins related to oxidative phosphorylation and iron storage. It is stored primarily in hepatocytes as ferritin, a cytosolic protein that stores non-heme iron in the form of ferrihydrite phosphate ([FeOOH]8 [FeOPO3H2]) to protect cells from its accumulation in the free form [Knovich et al., 2009; Wong, 2017]. Excess iron could cause toxicity problems due to its ability to act as a prooxidant agent, by generating reactive species that can damage DNA and proteins [Knovich et al., 2009]. Excessive dietary iron intake has been identified as a risk factor of colorectal cancer through population and animal studies [Chua et al., 2010]. Liver is an especially rich source of non-heme iron, although it also contains a percentage of heme iron. In pork liver, only 20-25% of the iron present is found as heme iron, while in pork loin it accounts for ~66% [Kongkachuichai et al., 2002]. However, in absolute values, the heme-iron content of liver can be considerably higher than that of meat [Kongkachuichai et al., 2002]. This is important because the human body absorbs heme iron more easily than non-heme one. In particular, the intestines absorb about 20-30% of heme iron while only 7% of non-heme [Mulvihill, 2014; SantéLhoutellier, 2014]. However, it should be taken into account that calcium inhibits the mucosal absorption of heme iron [Roughead et al., 2005].
Zn is an essential micronutrient required in many enzymes that participates in a multitude of basic biochemical and physiological processes in the cells of the human body [Grüngreiff et al., 2016; Tapiero et al., 2003]. Liver is the main organ involved in Zn metabolism, but it is mainly found in muscle and bone (~85% of body’s stores), while the liver accounts for ~5% [Grüngreiff et al., 2016]. Despite that, pork liver is by far one of the richest foods in Zn, even compared to livers from other animal species [Aoyagi et al., 1995; Stasiak et al., 2017].
Cu is also an essential micronutrient that acts as an important catalytic cofactor in redox reactions involved in fundamental biological functions of the human body [Tapiero et al., 2003]. Its main function is as a component of enzymes involved in iron metabolism [Tomović et al., 2019]. Pork liver acts as a Cu reservoir, which makes it a very rich source of this element [Keller, 2019; Tomović et al., 2019]. However, as happened with other monogastric mammalian livers, pork liver Cu has very low bioavailability [Aoyagi et al., 1995]; on the contrary, bioavailability of Cu is relatively high in avian and ruminant livers [Aoyagi et al., 1993]. It has been hypothesised that Zn may inhibit Cu utilisation, although the results are inconclusive [Aoyagi et al., 1995]. In addition, high intakes of Cu or Zn are known to interfere with the tissue utilisation and storage of Fe [Tapiero et al., 2003].
Pork liver is also a major source of Mn in the human diet. This element is responsible for the proper functioning of the nervous system, and is a component of the enzymes involved in the digestion and absorption of carbohydrates, lipids, and proteins. The results obtained in the present study regarding Mn content in pork liver (Table 2) are in good agreement with those reported by Stasiak et al. [2017]. According to these authors, pork liver is richer in Mn than muscle tissue, where it was completely missing.
CONCLUSIONS
The pork livers derived from the animal’s slaughter have a good microbiological quality, which makes them suitable for their valorisation within the food industry itself. The DSC thermograms partially reflect the chemical complexity of the liver and the thermostability of some of its components. The solubility profile of its protein fraction as a function of pH in the tested range (4.5 to 8.5) allows speculating that relatively high extraction yields can be obtained under basic conditions. This, along with the high protein content of the liver and the fact that only a small fraction of it corresponds to collagen suggests that obtaining protein extracts would be a good alternative for the valorisation of pork liver, especially if it is shown that they present interesting techno-functional properties. However, liver pH, water content and nutritional richness make it particularly susceptible to microbiological spoilage. A rapid processing at low temperatures and in the most hygienic conditions possible or, alternatively, its rapid freezing until the moment of use would be the most recommended in terms of food safety. These guidelines would also minimise oxidation phenomena, both of the lipid fraction and of some mineral elements, particularly iron due to its abundance and its effects on colour. While this would probably not have too much effect on the liver due to its own colour properties, it could strongly affect the colour of the extracts obtained.