INTRODUCTION
Diabetes mellitus (DM) is a chronic metabolic disorder resulting from inadequate insulin production by the pancreas or ineffective utilization of the insulin produced. The International Diabetes Federation (IDF) reported a current global diabetic prevalence of 537 million individuals in 2021, with a projected increase to over 783 million by the year 2045 [Saeedi et al., 2019; Sun et al., 2022]. This trajectory of diabetic cases poses a substantial challenge to human health care systems worldwide. Pharmaceutical interventions like oral metformin, DPP-4 inhibitors, SGLT-2 inhibitors, GLP-1 agonists, and α-glucosidase inhibitors help to regulate blood glucose levels. However, prolonged usage of these drugs often leads to side effects and drug resistance [Tahrani et al., 2016].
Numerous research groups are currently focusing their efforts on investigating herbs and functional foods in order to find antidiabetic molecules with reduced side effects compared to modern pharmaceutical drugs [Ahn et al., 2011; Hussain et al., 2022; Shahid et al., 2023; Sharmin et al., 2013]. Recent studies have highlighted the remarkable antidiabetic potential of polysaccharides derived from natural sources [Ji et al., 2023]. Polysaccharides extracted from both traditional medicinal plants [Zheng et al., 2019] and commonly consumed foods including pumpkin [Zhang et al., 2013], legumes [Bai et al., 2020], some fruits (e.g., goji berry) [Zhu et al., 2013], and green tea [Wang et al., 2015] were used by researchers to manage the blood glucose in diabetic patients and animals or insulin secretion in cell lines. Polysaccharides, recognized as significant biological macromolecules, exhibit also other activities, including lipid reduction, induction of tumor cell apoptosis, immunomodulation, prebiotic effects and antioxidant properties [Kakar et al., 2020; Wang Y. et al., 2019; Zeng et al., 2020].
Centella asiatica (L.) Urban, a member of the Apiaceae (Umbelliferae) tropical plant family, which was identified by Carl Linnaeus, is a globally distributed herb renowned for its extensive traditional and medicinal applications [Biswas et al., 2021]. This botanical species is indigenous to South and Southeast Asian nations, including India, China, Sri Lanka, Indonesia, and Malaysia. It is also naturally abundant in South Africa and Madagascar [Hamid et al., 2002]. Traditionally, C. asiatica is recognized for its culinary usage in salads and juices. It has gained prominence in the global herbal market, primarily due to its multifaceted medicinal potential [Biswas et al., 2021]. Notably, previous research studies have explored the health-promoting properties of C. asiatica through extracts, polyherbal formulations, and purified compounds. However, no research study has investigated the potential bioactivity of polysaccharides derived from C. asiatica leaves. Therefore, the present study optimized the extraction process and investigated the composition, and antidiabetic potential of C asiatica leaves (CAL) crude polysaccharides, and explored the potential of C. asiatica polysaccharides as an antidiabetic functional food product to be used in the future.
MATERIAL AND METHODS
Chemicals and reagents
All chemicals used were of the highest available grade. Sodium carbonate, monosaccharide standards (glucose, fructose, galactose, arabinose, rhamnose, xylose, mannose), glucuronic and galacturonic acids, α-glucosidase, α-amylase, and soluble starch were procured from Shanghai McLean Biochemical Technology Co., Ltd. (Shanghai, China). 4-Nitrophenyl-α-D-glucopyranoside (PNPG) and 3,5-dinitrosalicylic acid (DNS) were obtained from Tokyo Chemical Industry (Tokyo, Japan). Acarbose and soluble starch were sourced from Beijing Biotopped Science & Technology Co., Ltd. (Beijing, China). Sodium hydroxide, trifluoroacetic acid (TFA), hydrochloric acid, 1-phenyl-3-methyl-5-pyrazolone (PMP), and phosphate buffered saline (PBS) were purchased from Nanjing Chemical Material Corp (Nanjing, China).
Sample preparation
Centella asiatica (L.) Urban leaves (CAL) were collected in October 2022 from the local farm in Semenyih, Selangor, Malaysia. The specimen of CAL was taxonomically identified, and its voucher was deposited at the herbarium of the Universiti Kebangsaan Malaysia, Bangi, Malaysia. The freshly collected leaves were dried (50°C), pulverized into a fine powder using a high-powered mill (Model SF-2000, Chinese Traditional Medicine Machine Works, Shanghai, China), and then sifted through a 40-mesh sieve. The materials were stored in a desiccator at ambient temperature until needed.
Optimization of extraction conditions
Single-factor experiment
For the extraction process of CAL powder, three critical factors were considered: liquid (water) to solid ratio (mL/g), extraction temperature (°C), and extraction time (min). Each factor was investigated at five distinct levels, precisely: extraction temperatures (A) at 60°C, 70°C, 80°C, 90°C and 100°C; extraction times (B) at 30 min, 40 min, 50 min, 60 min, and 70 min; and liquid-to-solid ratio (C) at 15:1, 20:1, 25:1, 30:1 and 35:1. The crude extract was separated from the residue by centrifugation (3,000×g, 20 min) and dried until a constant weight had been achieved. The extraction yield was calculated using the following formula (1):
Response surface methodology
Based on the results of single-factor experiments, a three-level model was employed, comprising 17 experimental runs designed using the response surface methodology (RSM) with Box-Behnken design (BBD) to optimize extraction conditions. The experimental configurations were generated using the Design Expert 12 (State-Ease Inc., Minneapolis, MN, USA) software. Regression analyses of the experimental data were conducted and a non-linear quadratic model was fitted using the following formula (2) [Su et al., 2022]:
where: Y is the estimated response, Xi and Xj are the independent variables, βij is the interaction term, βii is the quadratic coefficient, βi is the linear coefficient, and β0 is the intercept.Aqueous extract preparation
The aqueous extract of CAL was prepared under the conditions used the in single-factor experiment, giving the highest extraction yield, and at the same time as close as possible to those optimized by RSM, i.e., 50 g CAL powder was suspended in water at a ratio of 25:1 (v/w) and extracted for 60 min at 80°C. The mixture was than centrifuged, and supernatant was separated. The extraction process was conducted three times.
Preparation of polysaccharide fractions
The CAL aqueous extracts were treated with ethanol to obtain alcohol-insoluble residues (AIR). Solvent was sequentially added to the extract to achieve final concentrations of 50% (v/v), 70% (v/v) and then 90% (v/v). Mixtures were left to stand for 24 h at room temperature to precipitate AIRs from which, after deproteinization, polysaccharide fractions designated as P50, P70, and P90, respectively, were obtained. Protein was removed by the Sevag method [Yao et al., 2020]. The resulting AIRs and Sevag reagent (chloroform and butyl alcohol mixture, 4:1, v/v) were thoroughly mixed in a shaker, vigorously shaken for 20 min, centrifuged at 1,500×g for 10 min, and the supernatants were collected. The procedure was repeated five times. The deproteinized solutions were re-precipitated in anhydrous ethanol at ten times the solution volume. The precipitates were collected by centrifugation (3,000×g, 20 min) and air-dried at 50°C until a constant weight had been achieved. The dried precipitates – polysaccharide fractions – were then stored in a dryer until use. The yield of polysaccharide fractions was calculated using the following formula (3):
Determination of contents of total sugars, uronic acids, and proteins
Total sugar content in the CAL polysaccharide fractions (P50, P70 and P90) was determined by the phenol-sulfuric acid method using D-glucose as a standard, as described by Yue et al. [2022]. The absorbance of the reaction mixture was read at 492 nm using a plate reader (Biochrom EZ Read 800 Microplate Reader, Cambridge, UK). The uronic acid content of P50, P70, and P90 was determined by the carbazole sulfuric acid method [Li et al., 2007], using D-galacturonic acid as a reference substance. In addition, protein content was analyzed using the Bradford’s method, with bovine serum albumin serving as the reference [Bradford, 1976]. The contents of total sugars, uronic acids, and proteins were expressed in g per 100 g of CAL polysaccharide fraction.
Mineral composition analysis
The mineral composition of CAL polysaccharide fractions (P50 and P70). was determined using a flame atomic absorption spectrometer (GGX-600, Beijing Haiguang Instrument Co., Ltd., Beijing, China) following the method outlined by Santos et al. [2014]. CAL polysaccharide fraction samples (0.20 g) were digested overnight with 10 mL of mixed acids (nitric acid and perchloric acid in a ratio of 4:1, v/v) and then, at 120°C for 3 to 5 h until the solution became transparent and colorless. The digested solution was transferred to a 25-mL volumetric flask, 2 mL of concentrated hydrochloric acid was added, and finally the flask was filled up with distilled water. Then, the atomic absorption spectrometry measurements were performed. The contents of magnesium, manganese, copper, zinc, calcium, iron, and selenium were determined, and respective results were expressed in mg per kg of the polysaccharide fraction.
Monosaccharide composition analysis
High-performance liquid chromatography (HPLC) was used to determine the monosaccharide composition of the P50 and P70 according to a previously described method [Chen et al., 2018] with slight modifications. Initially, 20 mg of the fractions were hydrolyzed at 100°C for 6 h using 2 mL of a 4 M trifluoroacetic acid (TFA) solution. The hydrolysates were diluted with anhydrous ethanol, and the solvents were evaporated at 45°C under vacuum. A new portion of ethanol was added to the solids, and then evaporation was repeated. This procedure was repeated several times until the hydrolysates were neutral. After hydrolysis, the monosaccharides of the P50 and P70 were derivatized with 1-phenyl-3-methyl-5-pyrazolone (PMP). Briefly, the hydrolysates were mixed with 1 mL of a 0.5 M PMP solution in methanol and 0.5 mL of a 0.4 M NaOH solution and reacted at 80°C for 60 min. After centrifugation, 0.4 M HCl (0.5 mL) and distilled water were added to the supernatant. Chloroform was then used to remove the excess PMP from the supernatant. The aqueous phase was filtered through a 0.45-μm membrane and taken for HPLC analysis.
The composition of monosaccharides was analyzed using the Shimadzu HPLC system (Model 2010AHT, Kyoto, Japan). A UV-Vis detector (SPD-20A, Shimadzu) was employed with the wavelength set at 250 nm. The chromatographic column used was an Athena-C18 (4.6×250 mm, 5 μm, ANPEL Laboratory Technologies, Shanghai, China). Separation was done with a solvent flow rate of 1 mL/min at room temperature. Mobile phase consisted of a 0.05 M ammonium acetate buffer (A), and a mixture of acetonitrile and water in a ratio of 80:20 (v/v) (B). The injection volume was 10 μL. The monosaccharides were identified by comparing the retention times of the analyte peaks in the sample to those of known standards analyzed under the same chromatographic conditions. The relative content (%) of individual monosaccharides of CAL polysaccharide fractions was calculated based on peak areas.
Analysis of antidiabetic potential
The antidiabetic potential of CAL polysaccharide fractions was assessed based on the ability of the fraction compounds to inhibit α-amylase and α-glucosidase activity. The α-amylase inhibitory activity of the CAL polysaccharide fractions was determined by using the iodine-starch method, following the protocol described by Wu et al. [2020]. Briefly, 100 μL of CAL polysaccharide fraction solutions with different concentrations (1 to 5 mg/mL) were combined with an α-amylase solution (100 μL, 0.5 mg/mL) prepared in 0.2 M phosphate buffered saline (PBS, pH 6.9). After incubation at 37°C for 10 min, 200 μL of a 1% soluble starch solution in 0.2 M PBS (pH 6.9) was added. Following another 10-min incubation at 37°C, a 3,5-dinitrosalicylic acid (DNS) solution (200 μL) was introduced. The mixture was then heated at 100°C for 10 min to deactivate α-amylase. Subsequently, the mixture was diluted with 4 mL of distilled water, and the absorbance was measured at 540 nm using a microplate reader (SpectraMax M3, Molecular Devices, San Jose. CA, USA). Acarbose served as the positive control in this assay.
The α-amylase activity inhibition (%) by CAL polysaccharide fractions was calculated using the formula (4):
where Acl and Abl-cl are the absorbances of reaction mixtures giving 100% enzymatic activity and 0% enzymatic activity, respectively; Aspl and Aspl-cl are the absorbances of the reaction mixture with the CAL polysaccharide fraction and the CAL polysaccharide fraction itself, respectively.The inhibitory potential of CAL polysaccharide fractions against α-glucosidase was evaluated using a method described by Salahuddin et al. [2020] with adjustment. An aliquot of 200 μL of CAL polysaccharide fraction solutions (concentration in range of 1 to 5 mg/mL) was mixed with 1.2 mL of 0.1 M PBS (pH 6.8) and 200 μL of an α-glucosidase solution (0.24 U/mL in 0.1 M PBS, pH 6.8). The mixture was incubation at 37°C for 10 min, and then, 200 μL of a 2.5 mM 4-nitrophenyl-α-D-glucopyranoside (PNPG) solution in the same PBS was added. After incubation at 37°C for 10 min, 0.8 mL of a 0.2 M sodium carbonate solution, was pipetted in. The absorbance of the mixture was recorded at 405 nm. Acarbose served as the positive control. The α-glucosidase activity inhibition (%) by CAL polysaccharide fractions was calculated according to formula (4) given above.
Statistical analysis
Differences among values were assessed using one-way analysis of variance (ANOVA) in SPSS 23.0 (IBM SPSS, Armonk, NY: IBM Corp., USA), and graphing was performed using Origin2021 (OriginLab Corp., USA). Duncan’s multiple range test was employed for multiple comparisons. The results were presented as mean and standard deviation (SD). Statistical significance was set at a p-value less than 0.05. All measurements were conducted in triplicate.
RESULTS AND DISCUSSION
Single-factor experiment
The results of single-factor extraction revealed that the liquid- -to-solid ratio, extraction temperature, and extraction time significantly influenced the yield of CAL extraction. The optimal conditions were determined as a liquid-to-solid ratio of 25:1 (mL/g), an extraction temperature of 80°C, and an extraction time of 60 min (Figure 1). These moderate conditions of extraction time and temperature seem safe for preserving CAL polysaccharides. As previously documented, higher temperatures and extended extraction times may enhance polysaccharide yield; however, they may induce structural changes in polysaccharides [Su et al., 2022; Zhang et al., 2015].
Extraction optimization using response surface methodology
To optimize the extraction yield of CAL, an RSM optimization experiment was conducted with a three-factor, three-level design. Traditional approaches, such as orthogonal testing, are commonly used in similar studies; however, RSM mitigates random errors in experiments and enables continuous analysis of experimental levels, thereby generating a continuous predictive model [Cai et al., 2019; Su et al., 2022]. The experimental design of the present study, yielding a binary multiple equation (5) to model the relationship between the CAL extraction yield (Y) and the three influencing factors was as follows:
where: Y is extraction yield of CAL, A signifies the liquid-to-solid ratio, B denotes the extraction temperature, and C represents the extraction time.Optimal extraction parameters for CAL polysaccharides were identified as a liquid-to-solid ratio of 24.43:1 (mL/g), an extraction time of 60.76 minutes, and an extraction temperature of 83.31°C (Table 1). The optimization model was well fitted to experimental data, as indicated by an F-value of 66.84 (p<0.0001), a lack of fit value of 0.2973 (p>0.05), and a determination coefficient (R2) of 0.9885 (Table 2). The coefficient of variation (cv) was found to be 1.83%. Furthermore, response surface diagrams and contour maps were generated using a multiple quadratic regression model (Figure 2). The steepness of the response surface directly correlated with response sensitivity, and contour maps revealed the strength of interactions [Wang L. et al., 2019]. Notably, contour lines plotted for the liquid-to-solid ratio, extraction time, and temperature exhibited an oval pattern, indicating their significant interactions (Figure 2).
Table 1
Box-Behnken design including factors (liquid-to-solid ratio, temperature and time), and response (extraction yield) used to optimize the Centella asiatica leaves (CAL) extraction.
Table 2
Data of analysis of variance for a regression model used in response surface methodology (RSM) for Centella asiatica leaves (CAL) extraction.
Fraction yield, and contents of total sugars, uronic acids, and proteins
Following the CAL aqueous extraction, polysaccharide precipitation from crude extract was carried out, resulting in three polysaccharide fractions termed P50, P70, and P90. The results demonstrated significantly highest P50 fraction yield (14.31%), followed by P70 and P90, respectively (Table 3). Moreover, the contents of total sugars and uronic acids (68.01 and 24.28 g/100 g, respectively) were also found to be higher in the P50 fraction of CAL, followed by P70 and P90. It was noted that the P90 fraction had the lowest yield and contents of total sugars, uronic acids, and proteins. Therefore, this fraction was excluded, and only the P50 and P70 fractions were subjected to downstream experiments.
Table 3
Fraction yield and content of total sugars, uronic acids, and proteins in Centella asiatica leaves (CAL) polysaccharide fractions (P50, P70 and P90).
Mineral and monosaccharide composition
The results of mineral content determination demonstrated that the CAL polysaccharide fractions (P50 and P70) had a high level of calcium, followed by magnesium and a low level of copper, and selenium (Table 4). Overall, the mineral content in both fractions (P50 and P70) was found to be in the order of calcium > magnesium > manganese > zinc > iron > copper > selenium. The analysis of the mineral composition of food and related products is essential for comprehending their nutritional significance, as these elements play a pivotal role in maintaining the health of both humans and animals [Jomova et al., 2022]. Several minerals and trace elements have been recognized for their significance in antihyperglycemic effect of plants traditionally used in the treatment of diabetes. These include sodium, potassium, magnesium, calcium, iron, copper, zinc, and others [Gholamhoseinian et al., 2020]. Moreover, essential trace elements like zinc, magnesium, manganese, and chromium play roles in insulin synthesis and secretion, and their levels are influenced by diabetes [Asif, 2017]. The results suggest that the CAL polysaccharide fractions hold a significant nutritional value as they are rich in essential mineral elements, particularly magnesium, calcium, and manganese. Moreover, these elements may be linked to their potential anti-hyperglycemic effects.
Table 4
Content of minerals (mg/kg) in Centella asiatica leaves (CAL) polysaccharide fractions (P50 and P70).
The HPLC separation of monosaccharides derived from polysaccharides of P50 and P70 fractions are shown in Figure 3. Eight compounds were identified: mannose, rhamnose, glucuronic acid, galacturonic acid, glucose, galactose, xylose, and arabinose. Table 5 provides the monosaccharide relative contents of P50 and P70 fractions. In P50, the predominant monosaccharides were galacturonic acid (24.55%) and galactose (20.55%). Similarly, in P70, a higher share of galactose (16.15%) and galacturonic acid (15.93%) was found in the composition of monosaccharides. The monosaccharide composition of various plant extracts has been observed to impact their inhibition potential against α-amylase and α-glucosidase. For instance, polysaccharide fractions derived from sesame seed hulls, particularly those rich in rhamnose, glucose, glucuronic acid, and galacturonic acid, have caused significant inhibition of both enzymes [Darwish et al., 2023]. Deng et al. [2020] found that a polysaccharide from Chaenomeles speciosa seeds, composed of rhamnose, glucuronic acid, galacturonic acid, and arabinose, proved to be an effective inhibitor of α-amylase and α-glucosidase.
Figure 3
High-performance liquid chromatography (HPLC) separation of monosaccharides derived from polysaccharides of Centella asiatica leaf (CAL) fractions – P50 (A) and P70 (B).
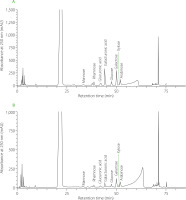
Table 5
Relative content (%) of monosaccharides derived from polysaccharides of Centella asiatica leaves (CAL) fractions (P50 and P70).
Antidiabetic potential
The key ones among the human enzymes responsible for the digestion of carbohydrates include glucosidase from the small intestine and pancreatic α-amylase. α-Amylase catalyzes the breakdown of long-chain carbohydrates, while α-glucosidase in the intestinal brush border is vital for oligosaccharide digestion [Hu et al., 2018]. The inhibition of α-amylase and α-glucosidase activities significantly impedes glucose conversion into bloodstream glucose, attenuating postprandial blood glucose levels.
The results of the present study demonstrated concentration-dependent inhibition of α-amylase activity by CAL polysaccharide fractions (Figure 4). Acarbose, the positive control, exhibited the highest inhibitory potential (82.7% at 5 mg/mL). Notably, the P50 fraction demonstrated the most substantial inhibitory effect at 5 mg/mL, yielding 68.3% inhibition, while the P70 fraction displayed a 46.8% inhibitory potential at the same concentration against α-amylase. A previous study by Wu et al. [2020] investigated polysaccharides from Rosa roxburghii Tratt leaves and reported half inhibitory concentrations (IC50) values of 10.16 mg/mL and 8.58 mg/mL for two polysaccharides, RLP-1.2 and RLP-2.1, respectively. Similarly, another relevant study by Fang et al. [2020] on Mentha haplocalyx polysaccharides reported an IC50 value of 11.65 mg/mL. Moreover, Schizophyllum commune polysaccharide, when tested at 10.0 mg/mL, exhibited 55.89% inhibition against α-amylase [Chen Z. et al., 2020]. Notably, our results surpassed these previous findings, indicating that P50 and P70 fractions may harbor active polysaccharides with potent α-amylase inhibitory properties.
Similar to the α-amylase inhibition potential, P50 and P70 also demonstrated concentration-dependent inhibition of α-glucosidase activity (Figure 4). Acarbose exhibited the highest inhibitory potential (81.7% at 5 mg/mL). Between CAL polysaccharide fractions, P50 showed a stronger inhibitory effect against α-glucosidase at 5 mg/mL (62.3%), than P70 (34.1%) (Figure 4). Wu et al. [2020] previously studied polysaccharides from Rosa roxburghii Tratt. leaves, reporting IC50 values for RLP-1.2 and RLP-2.1 against α-glucosidase at 6.29 mg/mL and 9.59 mg/mL, respectively. Another study on ginger stems and leaves reported an IC50 value of 7.66 mg/mL for polysaccharides obtained using hot water extraction [Chen X. et al., 2020]. The α-glucosidase inhibition activity of polysaccharides extracted from CAL has not been previously investigated, the results of the current study surpass those achieved in the aforementioned previous studies.
Figure 4
Inhibition of α-amylase (A) and α-glucosidase (B) activity by Centella asiatica leaf (CAL) polysaccharide fractions (P50 and P70). The results are presented as mean and standard deviation (n=3). ACB; acarbose.
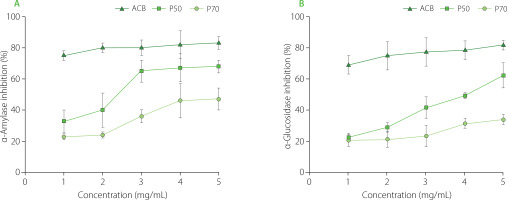
At each concentration (ranging from 1 to 5 mg/mL), P50 exhibited significantly higher inhibition of α-amylase and α-glucosidase compared to P70 (Figure 4). Previous research demonstrated that polysaccharides extracted from Hovenia dulcis (HDPs) using the accelerated solvent extraction technique (ASE-HDPs) exhibited greater α-amylase inhibitory activity than those extracted using the hot water method (HWE-HDPs), which was potentially attributed to the lower molecular weight of ASE-HDPs compared to HWE-HDPs [Yang et al., 2019]. Furthermore, the presence of hydroxyl and carboxyl groups on the branched chains of polysaccharides allows them to interact with amino acid residues of digestive enzymes, thereby reducing α-amylase activity [Nie et al., 2017]. Moreover, inorganic trace elements, such as zinc and manganese, were shown to exhibit antidiabetic properties, contributing to the efficacy of medicinal herbs [Lachkar et al., 2021]. Therefore, the elevated α-amylase and α-glucosidase inhibition activity observed in P50 could also be attributed to its higher content of elements such as zinc and manganese, as well as uronic acid.
Acarbose, used in the treatment of type 2 diabetes, may lead to side effects such as abdominal bloating due to its potent inhibitory activity on starch hydrolase, causing the accumulation of undigested carbohydrates [Deng et al., 2015]. These undigested carbohydrates can undergo fermentation by bacteria in the colon, resulting in the production of gas. Therefore, identifying a new inhibitor of α-amylase and α-glucosidase with a lower inhibitory activity than acarbose would be preferable. This study is the first to reveal the significant α-amylase- and α-glucosidase-inhibiting potential of CAL polysaccharides, suggesting their applicability as nutraceutical food ingredients for diabetes management.
CONCLUSIONS
In this study, the parameters for optimizing the extraction of crude polysaccharides from Centella asiatica leaves (CAL) were determined using the response surface methodology (RSM).
Subsequent analysis of the extracted polysaccharides revealed the presence of significant amounts of total sugars, with distinct variations in uronic acid and protein content among different fractions (P50, P70, and P90). The mineral composition demonstrated that CAL polysaccharide fractions had a high level of calcium, followed by magnesium and manganese. Among monosaccharides, galacturonic acid and galactose were dominant in the P50 and P70 fractions. Furthermore, both P50 and P70 fractions demonstrated concentration-dependent inhibition of α-amylase and α-glucosidase, suggesting their potential as nutraceutical food ingredients. Future studies could explore the underlying mechanisms of their antidiabetic effects and assess their safety and efficacy in in vivo models, paving the way for the development of novel and sustainable therapeutic interventions for diabetes.