INTRODUCTION
The food industry has recently been facing numerous challenges as consumers are looking for high-quality food products with health effects, such as antioxidant and antibacterial activity or prevention of cardiovascular diseases [Magnani et al., 2014]. Many epidemiological researches have revealed a positive correlation among the intake of food abounded in phenolic compounds and possible prevention and regulation of various disorders [Sato et al., 2011]. Dietary phenolics are secondary plant metabolites that are generally divided into two basic groups, i.e., flavonoids and non-flavonoids. Phenolic acids belong to the group of non-flavonoid phenolics which structures are characterized by phenol moiety stabilized by resonance and one carboxylic group [Kadar et al., 2021]. Caffeic acid (3,4-dihydroxycinnamic acid) is a representative of the group of cinnamic acid derivates that are also called phenylpropanoids and it is present in coffee drinks, berries, apples and other food sources [Magnani et al., 2014]. Gallic acid (3,4,5-trihydroxybenzoic acid) is composed of an aromatic ring with three hydroxyl groups and a carboxylic group. It is commonly present in the plant kingdom and found in free form or as a derivate in nuts, tea, grapes, berries, honey and other fruits and vegetables. Its ester derivates are used for the prevention of food spoilage and oxidative rancidity in food packaging materials, processed food and cosmetics [Badhani et al., 2015]. Among the many advantages of phenolic acids, these compounds are reported to have disadvantages related to sensitivity to processing and storage conditions, such as extreme temperatures, oxygen and light [Rashimi & Negi, 2020]. To improve the stability of phenolics, different encapsulation techniques are applied. Freeze-drying is a technique which is often deployed for encapsulation since it can protect thermo-sensitive components. For this purpose, different encapsulating polymers, such as polysaccharides, proteins or lipids, are used as carriers of bioactive compounds and in this way, the stability of phenolic properties can be maintained over a longer period. It was reported previously that dietary fibers have many beneficial impacts on gastrointestinal physiology, and also evoke a hypolipidemic impact since they can influence the expression of enzymes in lipid metabolism [Anderson et al., 2009]. Cellulose and its derivates can be applied as low-calorie substitutions for other carbohydrate additives, but also they can be used as carriers of flavor compounds and stabilizers of dispersed systems in different types of foods. They have also been demonstrated to act as delivery systems and protection carriers of different nutrients and active compounds in food products [Liu et al., 2017; Mu et al., 2014; Vukoja et al., 2021]. The majority of past investigations were organized to investigate on the one hand the release of phenolics from plant cell wall (PCW) components in the gastrointestinal tract and on the other hand the nature of the interaction between those compounds. Phenolics are allocated in the cell vacuoles where they can, during ripening, processing and food consumption, come into contact with PCW components and in this way their bioavailability can be affected [Padayachee et al., 2012a,b]. Cellulose, hemicellulose and pectin are the basic PCW components. The interactions of PCW components and phenolics cause the formation of the complex which can be beneficial in enhancing bioavailability of phenolics. Such complexes prevent the absorption of phenolics in the stomach or small intestine and assist in their transportation to the large intestine where fermentation is carried out with the help of intestinal bacteria [Padayachee et al., 2012b]. Generally, it has been concluded that the structural complexity and properties of phenolics and PCW components were the key elements in the assessment of bioaccessibility and bioavailability of phenolics. Non-covalent interactions (such as hydrogen and hydrophobic interactions) were the driving force between phenolics and PCW components, followed by environmental variables, such as pH and temperature [Le Bourvellec et al., 2004; Padayachee et al., 2012a,b; Phan et al., 2017; Renard et al., 2001; Tang et al., 2003]. Taking all this into consideration, cellulose has great potential to be used as a functional additive in the food products, such as beverages, dairy, fruit, meat and bakery products, hence, cellulose particles enriched with gallic and caffeic acid were formulated in this research.
The aim of this investigation was to formulate cellulose particles that are enriched with phenolic acids including gallic and caffeic acids and to investigate the impact of cellulose amount (2.5, 5, 7.5 and 10%, w/v) and time of complexation on the encapsulation of these phenolic acids onto cellulose. Stability of the formulated cellulose particles at ambient conditions over 12 months of storage in terms of retention of phenolic acids and antioxidant activity was also investigated.
MATERIALS AND METHODS
Chemicals
Microcrystalline cellulose and potassium persulfate were purchased from Kemika (Zagreb, Croatia). Gallic acid, caffeic acid, Trolox, 2,2-diphenyl-1-picrylhydrazyl (DPPH) radical, and 2,2′-azino-bis(3-ethylbenzothiazoline-6-sulfonic acid) diammonium salt (ABTS) were products of Sigma Aldrich (St. Louis, MO, USA). High-performance liquid chromatography (HPLC) grade methanol was bought from J.T. Baker (Deventer, Netherlands), and HPLC grade orthophosphoric acid (>85%) was from Fisher Scientific (Loughborough, UK). Cupric chloride, neocuproine and 2,4,6-tri(2-pyridyl)-s-triazine (TPTZ) were obtained from Acros Organic (Geel, Belgium), whereas hydrochloric acid (37%) was purchased from Carlo Erba Reagents (Sabadell, Spain).
Formulation of gallic acid/cellulose and caffeic acid/ cellulose particles
For the formulation of cellulose particles, cellulose (2.5, 5, 7.5 and 10%, w/v) was added into 50 mL of 5 mM gallic acid or caffeic acid solution in 10% (v/v) of ethanol. The obtained suspension was agitated at a magnetic stirrer (Stuart US152, Buch and Holm, Hervel, Denmark) at ambient temperature for 15 or 60 min. Afterwards, the mixtures were centrifuged at 2,545×g for 15 min to obtain precipitate, whereas the supernatant was discarded to eliminate non-adsorbed phenolic acids. The precipitate was frozen (−18°C) for 24 h and then freeze-dried in an Alpha 1-4 freeze dryer (Christ, Osterode am Harz, Germany). Sample freeze-drying conditions were as follows: the freezing temperature was adjusted to −55°C, the temperature of sublimation was adapted from −35 to 0°C at a vacuum level of 22 Pa, the isothermal desorption was adapted to 0–21°C at a vacuum level of 6 Pa. Part of the obtained particles were analyzed immediately, whereas the other part of the samples was sealed in plastic bags. The sealed samples were stored at ambient temperature for 12 months.
Extraction of phenolic acids from particles
For the extraction of phenolic acids, 0.1 g of each type of cellulose particles was weighed, then 5 mL of acidified methanol (ratio of HCl to methanol was 1:99, v/v) were added, and the mixture was well homogenized. After 24-h extraction at room temperature, the samples were filtrated, and clear extract was further analyzed. The extracts were analyzed for gallic acid and caffeic acid concentrations by HPLC, and used to determine antioxidant activity of the particles.
High-performance liquid chromatography of gallic and caffeic acids
The 1260 Infinity II HPLC system (Agilent Technologies, Santa Clara, CA, USA) was applied to determine gallic acid and caffeic acid contents in the prepared particles. The whole system contained a diode array detector (DAD), a quaternary pump, a vial sampler, and a Poroshell 120 EC C-18 column (4.6×100 mm, 2.7 µm). Orthophosphoric acid (0.1%, v/v) as a mobile phase solvent A and methanol (100%) as a mobile phase solvent B were used for chromatographic separation. Gallic acid was separated by isocratic elution with 10% of solvent A and 90% of solvent B for 6 min. For caffeic acid, the following gradient elution was applied: 0 min 5% B, 5 min 90% B, and 15 min 5% B. Operating condition were adjusted to the flow rate of 1 mL/min, a column temperature of 28°C and an injection volume of 10 μL. Stock solutions of gallic and caffeic acids in 100% methanol were applied to plot calibration curves in concentrations from 25 to 100 mg/L (r2=0.9967) and from 15 to 80 mg/L (r2=0.999), respectively. Peaks were recorded at 270 and 320 nm (for gallic acid and caffeic acid, respectively). Screenings were performed in duplicates, and results were expressed as g of phenolic acid per kg of cellulose particles (g/kg).
Determination of the antioxidant activity of particles
Antioxidant activity was determined using the assays with DPPH radicals (DPPH assay), ABTS cation radicals (ABTS assay), as well as ferric reducing antioxidant power (FRAP) and cupric ion reducing capability (CUPRAC) assays. The protocol of DPPH assay was defined elsewhere [Brand-Williams et al., 1995]. The DPPH radical solution was prepared and mixed in a glass tube with 0.2 mL of the extract. After 15 min in dark, absorbance of the mixture was read at 517 nm. The ABTS assay was conducted according to the procedure previously published by Arnao et al. [2001]. The ABTS radical cation solution and 0.2 mL of the extract were mixed, kept for 95 min in the dark, and their absorbance was read at 734 nm. The FRAP assay was performed as defined by Benzie & Strain [1996]. Briefly, the FRAP reagent was mixed with 0.2 mL of the extract in a glass tube. After 30 min, the absorbance of the mixture was read at 593 nm. The assay by Apak et al. [2004] was adapted in order to evaluate cupric reducing antioxidant activity. To conduct the analysis, 1 mL of CuCl2 solution (10 mM), 1 mL of neocuproine (7.5 mM), and 1 mL of ammonium acetate buffer (pH 7.0) were mixed with the sample and distilled water. The prepared mixture was left in the dark for 30 min, and afterwards absorbance was read at 450 nm. For all four assays, the calibration curves were plotted using Trolox as a standard, and the results were expressed as μmol of Trolox equivalent (TE) per kg of gallic acid/cellulose and caffeic acid/cellulose particles (μmol TE/kg). All measurements were done in triplicate using a Cary 60 UV/Vis spectrophotometer (Agilent Technologies).
Recording of infrared spectra
The infrared spectra of the samples were screened by the Cary 630 Fourier transform infrared – attenuated total reflectance (FTIR-ATR) spectrometer (Agilent Technologies) that contained the MicroLab Expert software. The screening of the cellulose and formulated particles was performed at the 4,000–600 cm−1 interval.
The following parameters were calculated as a ratio between band intensities: total crystalline index (TCI), lateral order index (LOI), and hydrogen bond intensity (HBI) according to the following Equations (1–3):
where: A1,370-1,360 is band intensity in the range of 1,370–1,360 cm−1 and A2,900-2,890 is band intensity in the range of 2,900–2,890 cm−1. where: A1,430-1,420 is band intensity in the range of 1,430–1,420 cm−1 and A897 is band intensity at 897 cm−1. where: A3,340-3,330 is band intensity in the range of 3,340– –3,330/1315 cm−1 and A1,315 is band intensity at 1,315 cm−1.RESULTS AND DISCUSSION
Content of phenolic acids in cellulose particles
Cellulose particles were determined for the content of gallic or caffeic acids, and results after complexation are presented in Table 1. The one-year storage stability of cellulose particles was also evaluated. From the results, it can be concluded that the amount of cellulose used for complexation as well as complexation time affected the adsorption of both phenolic acids. Also, the affinity of cellulose towards selected phenolic acids was different. Generally, cellulose had a higher affinity for caffeic acid. Gallic acid/cellulose and caffeic acid/cellulose particles contained phenolic acids in amounts of 3.399 and 4.665 g/kg, respectively. The highest content of phenolic acids was determined in the cellulose particles prepared with the lowest amount of cellulose, i.e., 2.5% (w/v). For both types of particles, a decrease was observed in the content of phenolic acids with the increase of cellulose amount during complexation (regardless of complexation time), thus, the content of gallic acid in particles with 10% (w/v) of cellulose was 2.964 g/kg in comparison to content of caffeic acid of 3.493 g/kg. Extended complexation did not have a positive effect on the adsorption of phenolic acids. Comparing particles with the highest content of phenolic acids, the same (p>0.05) content of gallic acid was determined after extended complexation (60 min) as after 15 min of complexation. For caffeic acid, a different trend was observed, so its content in the particles was significantly (p≤0.05) lower after extended complexation. The stability of particles was also evaluated. Gallic acid/cellulose particles were more stable over the 12 months of storage at ambient temperature than caffeic acid/cellulose particles. After storage, the content of gallic acid in cellulose particles slightly decreased (up to 3%), whereas that of caffeic acid decreased from 10 to 20%.
Table 1
Content (g/kg) of gallic acid and caffeic acid in gallic acid/cellulose (GA/C) and caffeic acid/cellulose (CA/C) particles, respectively, prepared by 15-min or 60-min complexation using different amounts of cellulose and stored for 12 months.
[i] Numbers in the particle codes (2.5, 5, 7.5 and 10) indicate the amount (%, w/v) of cellulose used for complexation. Values in the same column, separately for particles with gallic and caffeic acid, marked with different letters (a–d) are significantly different at p≤0.05. Values in the same raw, separately for particles before and after storage, marked with different letters (x, y) are significantly different at p≤0.05.
Interactions between different fibers, including cellulose, and phenolics depend on the molecular structure, physical properties and initial concentration of components used for complexation. It was pointed out that the main mechanism of their interactions was of non-covalent nature, like hydrogen bonds and hydrophobic interaction. Those interactions are also strongly affected by environmental conditions, like pH and temperature and time of complexation [Le Bourvellec et al., 2004; Padayachee et al., 2012a,b; Phan et al., 2017; Renard et al., 2001; Tang et al., 2003]. The main drivers of interactions between cellulose and phenolic acids are the molecular size and structure of phenolic acids [Le Bourvellec et al., 2004]. The basic structural characteristic of all phenolics is the presence of one or more benzene rings and hydroxyl groups by which they bind to specific sites on polysaccharide molecules. Generally, phenolics and fibers bind to each other throughout hydrogen bonds (formed between the OH groups of phenolic compounds and oxygen atoms of polysaccharides), as well as hydrophobic and covalent bonding (between phenolic acids and polysaccharides). Additionally, porosity, particle size and surface properties of dietary fibers can be crucial in the interaction between fibers and phenolic compounds [Quiros-Sauceda et al., 2014; Saura-Calixto, 2011]. Phenolic acids that have more hydroxyl groups in their structure and additionally contain hydrophobic regions ensure hydrogen bonding, thus promoting stronger interaction with molecules of dietary fibers. Phenolic acids can interact by non-covalent interactions with neutral saccharides (xyloglucans) present in the cellulose structure [Padayachee et al., 2012b]. Gallic acid contains 3 hydroxyl groups, whereas caffeic acid has 2; hence, both phenolic acids are candidates for the formation of hydrogen bonds with cellulose. Time of complexation did not play significant part in the adsorption of selected phenolic acids onto cellulose. At the beginning of the interaction between phenolics and cellulose, hydroxyl groups bonded on the cellulose ribbons’ surface. After initial interaction, hydrogen bonding and hydrophobic interaction occurred [Liu et al., 2017]. Extended complexation time, which included stirring, did not cause an increase in the adsorption of phenolic acids probably due to the breaking of hydrogen bonds and hydrophobic interactions. Additionally, it is possible that the formation of hydrogen bonds between cellulose molecules occurred due to the higher amount of cellulose during complexation, thereby decreasing the number of free binding sites for phenolic acids. A study on the binding capacity of diverse phenolics and cellulose revealed that binding was affected by the molecular structure of phenolics and it was in the range between 0.4 and 1.4 g/g of cellulose [Phan et al., 2015]. Interaction between cellulose and phenolics (gallic acid, caffeic acid, catechin, ferulic acid, chlorogenic acid, cyanidin 3-glucoside) happened within 1 min and quickly increased over 30 min. Afterwards, the binding between phenolics and cell wall polysaccharides decelerated [Padayachee et al., 2012a; Phan et al., 2015]. Probably, there are specific sites on the cellulose molecular structure that are responsible for the formation of strong biding regions. The second alternative is that cellulose has a certain degree of binding for phenolic molecules and above that degree, phenolic compounds are starting to make the ionic barrier for further binding [Padayachee et al., 2012a]. Our previous study also supported this biding tendency. Cellulose/raspberry particles prepared with 2.5% (w/v) of cellulose had the highest content of total phenolics. Higher adsorption of phenolics was achieved in samples prepared throughout 15 min of complexation than in those prepared for 60 min [Vukoja et al., 2021]. Also, our previous study, in which we investigated encapsulation of gallic acid onto pectin, showed that a higher content of pectin negatively affected encapsulation of gallic acid [Buljeta et al., 2022c].
Antioxidant activity of cellulose particles
Antioxidant activity of cellulose particles was evaluated by four assays, namely DPPH, ABTS, FRAP and CUPRAC, that are the most common for evaluation of this parameter. Results are presented in Tables 2, 3, 4 and 5. Different values of antioxidant activities were obtained depending on the applied assay, i.e., its mechanism of action. Antioxidant activity obtained by the DPPH assay for gallic acid/cellulose particles before storage ranged from 391.6 to 419.4 μmol TE/kg and the values for particles prepared with 2.5, 5 and 7.5% (w/v) of cellulose for each complexation time did not differ significantly (p>0.05), indicating there was no considerable variation in DPPH radical scavenging activity among the particles (Table 2) even though there was a difference in gallic acid content (Table 1). In the stored particles, a slight change of DPPH radical scavenging activity was noted, and the same trend was retained as before storage (Table 2). Results of antioxidant activity determination for caffeic acid/ cellulose particles obtained by the DPPH assay followed the trend that was obtained for caffeic acid content in these particles before and after storage. In the ABTS assay, values of antioxidant activity ranged from 478.6 to 589.7 μmol TE/kg and 874.7 to 1,691.0 μmol TE/kg for gallic acid/cellulose and caffeic acid/ cellulose particles before storage, respectively (Table 3). By comparison of the results of the ABTS assay of the samples prepared with the same cellulose amount and different complexation times, it was observed that a complexation time of 15 min was more favorable for the samples of gallic acid with 2.5 and 10% (w/v) of cellulose, whereas for the samples with caffeic acid, this effect was observed for the samples prepared with 2.5 and 5% (w/v) of cellulose. A complexation time of 60 min had a positive effect only on the gallic acid sample prepared with 5% (w/v) cellulose. ABTS radical cation scavenging activity followed the trend of phenolic acid content before and after storage. The results of the FRAP assay of the particles before storage ranged from 134.8 to 163.0 μmol TE/kg and 48.9 to 71.7 μmol TE/kg for gallic acid/cellulose and caffeic acid/cellulose particles, respectively (Table 4). Interestingly, in this assay, the highest antioxidant activity was determined for the gallic acid/cellulose particles, whereas in DPPH and ABTS assays the highest values were obtained for the caffeic acid/cellulose particles. Additionally, regarding FRAP (as it was the case with DPPH radical scavenging activity), there was not so large difference among the particles with different cellulose amounts even though there was a difference in phenolic acid content. The results obtained by CUPRAC assay followed the trend observed for the content of phenolic acids in cellulose particles (Table 5). These results ranged from 1,963.4 to 2,635.5 μmol TE/kg for gallic acid/cellulose and from 1,777.3 to 2,646.3 μmol TE/kg for caffeic acid/cellulose particles before storage. The highest value of antioxidant activity determined for the samples with gallic acid by the CUPRAC assay before storage was noted for the samples with 2.5% (w/v) cellulose regardless of complexation time, whereas 15 min of complexation and 2.5% (w/v) cellulose caused the highest antioxidant activity of the samples with caffeic acid.
Table 2
DPPH radical scavenging activity (μmol TE/kg) of gallic acid/cellulose (GA/C) and caffeic acid/cellulose (CA/C) particles prepared by 15-min or 60-min complexation, after complexation using different amounts of cellulose and stored for 12 months.
[i] Numbers in the particle codes (2.5, 5, 7.5 and 10) indicate the amount (%, w/v) of cellulose used for complexation. Values in the same column, separately for particles with gallic and caffeic acid, marked with different letters (a–d) are significantly different at p≤0.05. Values in the same raw, separately for particles before and after storage, marked with different letters (x, y) are significantly different at p≤0.05. TE, Trolox equivalent.
Table 3
ABTS radical cation scavenging activity (μmol TE/kg) of gallic acid/cellulose (GA/C) and caffeic acid/cellulose (CA/C) particles prepared by 15-min or 60-min complexation using different amounts of cellulose and stored for 12 months.
[i] Numbers in the particle codes (2.5, 5, 7.5 and 10) indicate the amount (%, w/v) of cellulose used for complexation. Values in the same column, separately for particles with gallic and caffeic acid, marked with different letters (a–d) are significantly different at p≤0.05. Values in the same raw, separately for particles before and after storage, marked with different letters (x, y) are significantly different at p≤0.05. TE, Trolox equivalent.
Table 4
Ferric reducing antioxidant power (μmol TE/kg) of gallic acid/cellulose (GA/C) and caffeic acid/cellulose (CA/C) particles prepared by 15-min or 60-min of complexation using different amounts of cellulose and stored for 12 months.
[i] Numbers in the particle codes (2.5, 5, 7.5 and 10) indicate the amount (%, w/v) of cellulose used for complexation. Values in the same column, separately for particles with gallic and caffeic acid, marked with different letters (a–d) are significantly different at p≤0.05. Values in the same raw, separately for particles before and after storage, marked with different letters (x, y) are significantly different at p≤0.05. TE, Trolox equivalent.
Table 5
Cupric reducing antioxidant capacity (μmol TE/kg) of gallic acid/cellulose (GA/C) and caffeic acid/cellulose (CA/C) particles prepared by 15-min or 60-min of complexation using different amounts of cellulose and stored for 12 months.
[i] Numbers in the particle codes (2.5, 5, 7.5 and 10) indicate the amount (%, w/v) of cellulose used for complexation. Values in the same column, separately for particles with gallic and caffeic acid, marked with different letters (a–d) are significantly different at p≤0.05. Values in the same raw, separately for particles before and after storage, marked with different letters (x, y) are significantly different at p≤0.05. TE, Trolox equivalent.
Throughout our results, it was evident that different values of antioxidant activity were achieved by the application of different assays. Antioxidant activity is one of the many biological activities of phenolics and it is highly dependent on their chemical structure. Two mechanisms are involved in their antioxidant effects and those are single electron transfer (SET) and hydrogen atom transfer (HAT) [Chaillou & Nazareno, 2006; Mercado-Mercado et al., 2020]. The antioxidant activity of phenolic acids is improved with an increased number of methoxy groups, but especially hydroxyl groups, in their structures [López-Martínez et al., 2015]. Craft et al. [2012] also stated that polyphenols with an electron donor group attached to the aromatic ring had considerable antioxidant activity. By studying the chemical structure of gallic acid it can be observed that para O–H bond has the lowest binding energy; thus, it is the weakest bond present in its structure and exposed to the reaction with free radicals [Uranga et al., 2016]. Kikuzaki et al. [2002] investigated antioxidant activity of caffeic acid and its related compounds (p-coumaric, ferulic and sinapic acid). The obtained results showed that caffeic acid exhibited high antioxidant activity determined by the DPPH assay. It is known that the number of hydroxyl groups attached to the benzene ring and the ortho substitution with the electron donor methoxy group that caused increased stability of the phenoxy radical affected positively the antioxidant effect of hydroxycinnamic acids (such as caffeic acid) on DPPH radical [Kikuzaki et al., 2002]. Theoretical results obtained in the study by Uranga et al. [2016] showed that antioxidant activity of hydroxycinnamic acids, such as caffeic and chlorogenic acid, appeared through SET reaction in DPPH and FRAP assays. Except for the number and position of OH groups attached to the aromatic ring that determine the antioxidant activity of the phenolic acids, it was suggested that hydroxycinnamic acids possessed higher antioxidant potential than hydroxybenzoic acids probably due to the –CH=CH–COOH group which has a better ability to donate H atoms and stabilize radicals than the –COOH group [Mercado-Mercado et al., 2020]. There is an exception for the DPPH assay, where hydroxybenzoic acids, such as gallic acid, show higher activity. In the reaction of phenolic acids with radicals, a quinone derivative may be produced or the original chemical structure can be preserved. Results of a study conducted by López-Martínez et al. [2015] showed that caffeic acid formed quinones, whereas gallic acid preserved its structure and managed to neutralize DPPH. Comparing DPPH radicals and ABTS radical cations, DPPH radicals have higher selectivity in reaction with donors of hydrogen than ABTS radical cations [Loncaric et al., 2014]. Apak et al. [2004] stated that, additionally to conjugation of the molecule, the number and position of OH groups are directly connected to the antioxidant activity in the CUPRAC method. The binding of phenolic acids on the cellulose probably changed the number of available hydroxyl groups on phenolic acids and also caused changes in reaction with DPPH radicals and ABTS radical cations as well as the reduction of metal ions in the other two assays.
DPPH commonly reacts through SET reactions, but sometimes DPPH radical can be neutralized by either HAT or SET mechanisms as well as unrelated reactions [Craft et al., 2012; Prior et al., 2005]. The DPPH radical scavenging assay is one of the most often used analysis for the determination of antioxidant activity. DPPH is a commercially available stable chromogen radical and it does not need to be generated before the analysis [Shahidi & Zhong, 2015]. In the ABTS assay, an intensely colored ABTS radical cation is formed, and the antioxidant effect is measured as the ability of the compounds to decrease the color reacting with the radical [Prior et al.,2005]. In turn, the FRAP assay measures the reduction of the ferric ion (Fe3+)-ligand complex to the blue-colored ferrous (Fe2+) complex by the action of antioxidant [Shahidi & Zhong, 2015]. In our study, tripyridyltriazine (TPTZ) was used as the iron-binding ligand. The FRAP assay is simple, robust and cheap, and is based on SET mechanism; hence, it is recommended to be used in combination with other assays to check the dominant mechanism for different antioxidants [Prior et al.,2005]. Finally, the CUPRAC assay is based on the reduction of cupric (Cu2+) to cuprous ion (Cu+) using antioxidants [Prior et al.,2005]. Analogous to the FRAP assay, a ligand (neocuproine) forms a copper-ligand complex to facilitate absorbance measurement in the CUPRAC assay [Shahidi & Zhong, 2015].
Infrared spectra of cellulose particles
FTIR spectra of cellulose, gallic acid/cellulose particles and caffeic acid/cellulose particles are presented in Figure 1. FTIR spectra of cellulose are in accordance with the spectra obtained by Cichosz & Masek [2002]. The band observed in the region from 3,660 to 2,900 cm−1 is connected with the stretching vibrations of O–H and C–H bonds which are present in polysaccharide molecules [Cichosz & Masek, 2020; Hospodarova et al., 2018; Poletto et al., 2011; Popescu et al., 2011; Rosa et al., 2010]. This region was also identified in all our samples but there was a difference in spectra intensity. In fact, the whole IR spectra of gallic acid/ cellulose and caffeic acid/cellulose particles had higher intensity than these of cellulose, which could be related to the adsorption of phenolic acids onto cellulose. Caffeic acid/cellulose particles had a higher content of this phenolic acid and higher intensity in comparison to the gallic acid/cellulose particles. The broad band at 3,300 cm−1 was connected to the stretching vibration of the OH groups in polysaccharides with inter- and intra-molecular vibrations of hydrogen bonds in cellulose [Poletto et al., 2011; Popescu et al., 2011; Rosa et al., 2010]. Generally, the band at 2,894 cm−1 corresponded to CH stretching vibrations of all hydrogen carbon constituents in polysaccharides [Poletto et al., 2011; Popescu et al., 2011]. This band was more pronounced in the case of the tested particles compared to cellulose. Next to the already mentioned region (associated with the formation of hydrogen bonds), another two interesting regions of cellulose are 1,430–1,420 cm−1 and 900–890 cm−1. The first one is associated with the amount of the crystalline structure of the cellulose and the second one with the amorphous fraction [Jonoobi et al., 2010; Hospodarova et al., 2018]. Additionally, the following parameters have been calculated: total crystalline index (TCI), lateral order index (LOI) and hydrogen bond intensity (HBI), to allow the interpretation of cellulose structure and changes occurring on it, and respective results are presented in Table 6. HBI increased with the adsorption of phenolic acids on cellulose. For caffeic acid/cellulose particles, this increase was from 1.00 to 1.35, and for gallic acid/cellulose particles from 1.00 to 1.25. This rise could be interpreted by the formation of hydrogen bonds [Cichosz & Masek, 2020]. However, these hydrogen bonds could also be a consequence of cellulose-cellulose interactions. Since TCI parameter decreased with the adsorption of phenolic acids, cellulose-cellulose interactions can be excluded and this proves binding of phenolic acids on cellulose through hydrogen bonds. TCI was 1.21 for cellulose, 1.08 for caffeic acid/cellulose particles, and 1.09 for gallic acid/cellulose particles. The number of OH groups with protons that can be involved in the formation of hydrogen bonds is lower in comparison to the number of oxygen atoms that are able to induced this type of interaction [Cichosz & Masek, 2020; Lindman et al., 2010]. Consequently, there are numerous options for molecules of specific structures to interact with cellulose molecules. Gallic and caffeic acids have phenolic OH groups and one carboxyl group; thus, their structure makes them possible to be involved in hydrogen bonding.
Figure 1
Infrared spectra of cellulose (C), gallic acid/cellulose particle (GA/C) and caffeic acid/cellulose particle (CA/C).
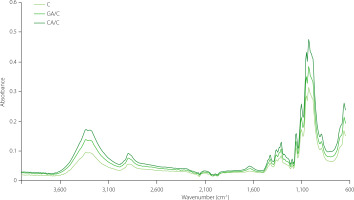
Table 6
Total crystalline index (TCI), lateral order index (LOI) and hydrogen bond intensity (HBI) of cellulose (C), gallic acid/cellulose (GA/C) and caffeic acid/cellulose (CA/C) particles.
Particle | TCI | LOI | HBI |
---|---|---|---|
C | 1.21 | 1.00 | 0.50 |
GA/C | 1.09 | 1.08 | 0.58 |
CA/C | 1.25 | 1.35 | 0.53 |
The FTIR analysis also supported the theory that hydrogen binding occurred between phenolic acids and cellulose. Other studies also proved that changes on IR spectra are visible upon the binding of phenolics on cellulose [Vukoja et al., 2021]. The binding of phenolics also revealed changes in the structure on other fibers [Abdelwahab et al., 2013; Buljeta et al., 2021, 2022a,b; Kopjar et al., 2022].
CONCLUSIONS
The aim of this research was to formulate functional cellulose particles enriched with phenolic acids (gallic or caffeic acid). Based on results obtained, it was concluded that cellulose had a higher affinity towards caffeic acid. The amount of cellulose during complexation affected the adsorption of both phenolic acids, and it was concluded that a decrease of the adsorption of phenolic acids occurred with the increase of cellulose amount. Results of the stability testing showed that gallic acid/ cellulose particles were more stable during one year of storage at ambient temperature. Considering antioxidant activity of the formulated particles, it was observed that it generally followed the content of phenolic acids on cellulose particles. The formulated cellulose particles can be used for the enrichment of different types of foods (fruit, dairy, and bakery) with cellulose and phenolic acids, additionally improving their oxidative stability. Consequently, these particles could offer a natural, plant-based way of controlling the nutritional value of foods and their stability.