ABBREVIATIONS
ALT, alanine aminotransferase; AST, aspartate aminotransferase; CPT1a, carnitine palmitoyltransferase 1a; CPT1b, carnitine palmitoyltransferase 1b; CVD, cardiovascular disease; FAS, fatty acid synthase; HDL-C, high-density lipoprotein cholesterol; HFD, high-fat diet; IL-1β, interleukin-1beta; IL-6, interleukin-6; LDL-C, low-density lipoprotein cholesterol; ND, normal diet; PPAR-α, peroxisome proliferator-activated receptor-alpha; PPAR-γ, peroxisome proliferator-activated receptor-gamma; PSO, perilla seed oil; PUFA, polyunsaturated fatty acids; qRT-PCR: quantitative real-time reverse-transcription PCR; SFA, saturated fatty acids; SFO, sunflower oil; TC, total cholesterol; TNF-α, tumor necrosis factor-alpha; TG, triglycerides; TSO, tea seed oil; UCP1, uncoupling protein 1; USFA, unsaturated fatty acids.
INTRODUCTION
Obesity is considered one of the most prevalent health problems worldwide, with an alarming increase in the number of obese people every year [Gregg & Shaw, 2017]. In recent years, obesity prevalence has more than doubled, with more than 1.9 billion overweight individuals globally, at least 600 million of whom were clinically obese [Zhang et al., 2019]. Previous studies have demonstrated that obesity can be caused by a variety of factors, including a high-fat diet (HFD), and that it is linked to a range of metabolic disorders, such as type 2 diabetes, dyslipidemia, non-alcoholic fatty liver disease, and cardiovascular disease (CVD) [Li & Ji, 2018; Parto & Lavie, 2017]. Indeed, the onset and development of metabolic disorders have been associated with increased production of pro-inflammatory hormones and cytokines, such as leptin, resistin, tumor necrosis factor-alpha (TNF-α), interleukin-6 (IL-6), and interleukin-1beta (IL-1β), together with a decrease in the production of anti-inflammatory adipokines such as adiponectin [Ouchi et al., 2011].
Inflammation-mediated pathways are likely to play an important role in metabolism regulation [Stienstra et al., 2012]. It has been shown that the peroxisome proliferator-activated receptor-alpha (PPAR-α) plays a crucial role in metabolism regulation, primarily through enhancing β-oxidation by increasing the expression of its associated genes [Takahashi et al., 2016]. Carnitine palmitoyltransferase 1 (CPT1), a PPAR target gene, is necessary for fatty acid transfer into the mitochondria for β-oxidation [Chen et al., 2017]. Additionally, several genes related to lipid metabolism are linked to adipose tissue inflammation. For example, PPAR-α activation can reduce inflammatory cytokine production by suppressing nuclear factor kappa B (NF-κB) signaling, whereas peroxisome proliferator-activated receptor gamma (PPAR-γ) activation can inhibit macrophage activation and the production of inflammatory cytokines, such as TNF-α, IL-1β, and IL-6 [Decara et al., 2020]. Furthermore, the uncoupling protein 1 gene (UCP1), found in the inner mitochondrial membrane, plays a crucial role in adipose tissue’s browning and affects lipid metabolism [Mishra et al., 2021]. Although UCP1 protein is abundant in brown adipose tissue, its presence in a white fat cell indicates that it has transformed into a light brown (beige) fat cell. Activation of brown and beige adipocytes can affect systemic energy metabolism, promoting substrate oxidation and energy expenditure, regardless of its use as a therapeutic target for many metabolic conditions, such as diabetes and obesity [Whitehead et al., 2021]. Therefore, regulating lipid metabolism and the expression of genes related to inflammation is a beneficial approach to alleviating obesity.
In recent years, vegetable oils have received much attention due to their health advantages and possible use as alternative drugs to treat various diseases. Epidemiological evidence indicates that n3 and n6 polyunsaturated fatty acids (PUFAs) and n9 monounsaturated fatty acids (MUFAs) can be used as an alternative to saturated fatty acids (SFAs) with additional health benefits [Bjermo et al., 2012]. Tea seed oil (TSO) is an edible vegetable oil produced from pressed ripe tea (Camellia oleifera) seeds and has a fatty acid composition similar to olive oil [Tung et al., 2019]. It is principally composed of oleic acid (78 to 86%), and the percentage of unsaturated fatty acids (USFAs) in TSO may be as high as 90%, which is the highest proportion of USFAs found in any edible oil to date [He et al., 2011]. Sunflower oil (SFO) is a popular edible vegetable oil produced from crushed sunflower seeds (Helianthus annuus) that is mainly used for frying and has a high content of oleic and linoleic acids. Adeleke & Babalola [2020] demonstrated that SFO provides several health benefits, including blood pressure control, cholesterol reduction, diabetes management, and many other health benefits. Perilla (Perilla frutescens) is a Lamiaceae family annual plant commonly grown in most Asian nations, including South Korea, China, and Japan, and is recognized to confer many health benefits [Thomas et al., 2020b]. Perilla seed oil (PSO) is a good source of n3 PUFAs, particularly α-linolenic acid, and has numerous health benefits such as anti-inflammatory, anti-oxidant, and anti-obesity effects [Asif, 2011]. Though many different oils are used in our daily lives, only a few studies have compared the health effects of oils with different fatty acid (FA) compositions.
Therefore, the aim of this study was to assess the effects of PSO, SFO, and TSO on mice with HFD-induced obesity. The biochemical and physiological indicators of obesity-associated disorders were examined to evaluate the impact of the three studied oils. Furthermore, quantitative real-time reverse-transcription PCR (qRT-PCR) was used to explore the molecular mechanisms of tested oils against obesity. The findings of this study could contribute to the exploration and development of safe and effective dietary supplements for obesity treatment.
MATERIALS AND METHODS
Materials and reagents
Tea (Camellia oleifera) seeds were obtained from Chongqing Amber Tea Oil Co., Ltd. (Youyang, Chongqing, China). Perilla (Perilla frutescens) seeds were purchased from Chongqing Qingzhong Road Perilla Planting Professional Cooperative (Pengshui, Chongqing, China). Sunflower (Helianthus annuus) seeds were obtained from the biggest local supermarkets (Beibei, Chongqing, China). The following PCR primers were purchased from Sangon Biotech (Shanghai) Co., Ltd. (Shanghai, China): glyceraldehyde 3-phosphate dehydrogenase (GAPDH), leptin, adiponectin, TNF-α, IL-1β, IL-6, PPAR-α, PPAR-γ, fatty acid synthase (FAS), carnitine palmitoyltransferase 1a (CPT1a), carnitine palmitoyltransferase 1b (CPT1b), and UCP1. All chemicals and reagents used were analytical grade and bought from commercial sources.
Seed oil extraction
The cold pressing method was used in the lab for crushing the seeds and extracting the oil using a screw press machine. The seeds were pressed at room temperature with a temperature of 50±10°C inside the press, and the resulting oil temperature was 39±1°C. Next, the oil was clarified by centrifugation at 5,000 rpm for 15 min (desktop refrigerated centrifuge 5810R, Eppendorf Ltd., Hamburg, Germany) and filtered through fine cheesecloth. The oil samples were stored at 4°C in dark-colored glass vials until further use.
Fatty acid composition analysis
The FA profile was determined using gas chromatography after conversion to fatty acid methyl esters (FAMEs) [Huang et al., 2015]. The FAMEs were obtained by mixing 100 mg of oil and 0.2 mL of 2 M methanolic potassium hydroxide solution with 2 mL of n-hexane at 50°C for 15 min. The gas chromatograph was a Shimadzu GC-2010 plus (Shimadzu, Tokyo, Japan) with a flame ionization detector and a DB-FFAP silica capillary column (30 m × 0.53 mm; film thickness 0.50 m) attached. As a carrier gas, nitrogen (flowing rate, 10 mL/min) was used, and the analysis was designed as follows: for 1 min, maintain a temperature of 50°C, the temperature was then raised at a rate of 15°C/min to 170°C and then at a rate of 2°C/min from 170 to 250°C; the temperature was maintained at 250°C for the next 10 min. The detector and injector temperatures were set at 270°C and 230°C, respectively. The concentration of each FAME was calculated by the area under the peak using ChemStation software (Agilent Technologies Inc., Wilmington, DE, USA). The results were expressed as a percentage of the total FAs.
Animal experimental design
Fifty healthy male Kunming (KM) mice (7-weeks old, weight 30±2 g) were obtained from Jiangsu Xietong Pharmaceutical Bio-engineering Co., Ltd., Nanjing, China (quality certificate number: SCXK (XIANG) 2019-0004). All mice were housed in a specific pathogen-free (SPF) barrier laboratory of the College of Pharmaceutical Sciences, Southwest University (facility license number: SYXK (YU) 2020-0006) in a controlled laboratory environment (12 h light/dark cycle, 25±2°C temperature, and 55±5% relative humidity) with free access to food and water throughout the experiment.
After a week of acclimation, the mice were randomly divided into five groups (n=10 per group): a control group (ND) fed a standard diet (contains 10% fat, 3.85 kcal/g, Jiangsu Xietong Pharmaceutical Bio-engineering Co., Ltd., Nanjing, China); HFD (model group) fed a HFD (contains 60% fat, 5.26 kcal/g, Jiangsu Xietong Pharmaceutical Bio-engineering Co., Ltd., Nanjing, China); PSO group; SFO group; TSO group (PSO, SFO, and TSO groups were fed with the same HFD but supplemented with 2 g/kg body weight per day with PSO, SFO, and TSO, respectively) by oral gavage for 8 consecutive weeks. The composition of ND and HFD is shown in Table 1. Mice in the ND and HFD groups were administered the same dose of saline solution to create the same treatment stress (gavage procedure). All experimental animal procedures were approved and supervised by the Institutional Animal Care and Use Committee (IACUC) of Southwest University, Chongqing, China (IACUC No. approved: IACUC-20210225-06) following the legislation and regulations governing the care and use of laboratory animals. The experimental design is shown in Figure 1A.
Figure 1
Perilla seed oil (PSO), sunflower oil (SFO), and tea seed oil (TSO) supplementation reduced body weight gain but not food intake in HFD-induced obese mice. (A) Scheme of the study design; (B) Body weight growth of mice consuming the specified diets for the 8 weeks; (C) Body weight gain of mice at the end of the study period; (D) Food intake of mice during the study period; (E) Calorie intake of mice during the study period; (F) Food efficiency ratio (FER) calculated as body weight gain (g/day)/food intake (g/day)×100 of mice after 8 weeks of food intervention. ND, mice fed a normal diet; HFD, mice fed a high-fat diet; PSO, SFO, and TSO, mice fed a high-fat diet supplemented with PSO, SFO, and TSO at 2 g/kg body weight per day, respectively. Data are presented as mean ± standard deviation (n=8). Means with different letters (a–c) were considered significantly different at p<0.05, according to Tukey’s test.
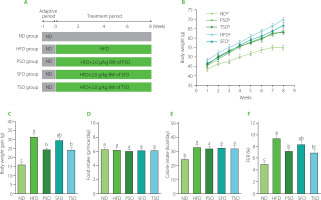
Table 1
Compositions of normal and high-fat diets.
Dosage information
The dietary oil doses were chosen based on previous studies from our group and others using different oils [Cheng et al., 2014; Hirabara et al., 2013; Huang et al., 2021; Wang et al., 2020]. They were calculated based on body surface area, as reported by Reagan-Shaw et al. [2008]. According to this suggestion, a daily oil intake of 2 g/kg body weight per day in mice is precisely equivalent to 162 mg/kg body weight per day in humans (i.e., 9.72 g per day for a 60 kg individual), which is markedly less than the daily intake recommended by the Chinese Nutrition Society (25–30 g per day) [Wang et al., 2016].
Measurement of body weight and food consumption
At the initiation of the experiment and at the end of each other week, body weight was measured. A sensitive electronic scale was used to weigh an empty scoop container. Then, the mice were placed inside the container and weighed individually. The weight of each mouse was calculated as the difference in weight between the empty container and the container with the mouse inside. Furthermore, throughout the experiment, food weight was measured daily in a container of known weight and weighed using an electronic weighing balance. The food weight was obtained from the weight difference between the empty container and the container with the food inside. The food consumption was calculated by subtracting the remaining food weight from the served food weight.
Sample collection
After the end of the experiment, all mice were fasted overnight, and blood samples were taken by uprooting eyeballs after light narcotizing with ether (1.90%) for serum preparation. The serum was obtained after blood clotting by centrifugation at 3,500 rpm for 15 min at 4°C and then stored at −80°C until use. The mice were then executed by cervical dislocation, and the organs and adipose tissue, including the liver, kidney, lung, spleen, perirenal adipose tissue (PAT), and epididymal adipose tissue (EAT), were excised, weighed separately, and stored at −80°C until analysis.
Serum biochemical analysis
The biochemical analysis kits for insulin (cat. no. H203-1-2), glucose (cat. no. A154-1-1), total cholesterol (TC; cat. no. A111-1-1), triglycerides (TG; cat. no. A110-1-1), high-density lipoprotein cholesterol (HDL-C; cat. no. A112-1-1), low-density lipoprotein cholesterol (LDL-C; cat. no. A113-1-1), alanine aminotransferase (ALT; cat. no. C009-2-1), and aspartate aminotransferase (AST; cat. no. C010-2-1) were used to measure the serum concentrations of insulin, glucose, TC, TG, HDL-C, LDL-C, ALT, and AST, according to the manufacturer’s instructions (Nanjing Jiancheng Biology Engineering Institute, Nanjing, China). The free fatty acid (FFA) levels were measured using the colorimetric method with a serum FFA kit (cat. no. E1001; Applygen Technologies Inc., Beijing, China). Moreover, very low-density lipoprotein cholesterol (vLDL-C) was calculated as TG/5 according to the Friedewald formula [Friedewald et al., 1972], while the atherogenic index was calculated by the Equation (1):
The homeostatic model assessment for insulin resistance (HOMA-IR) index and the homeostatic model assessment for insulin sensitivity (HOMA-IS) index were calculated as previously reported [Qu et al., 2019a].
ELISA analysis
The enzyme-linked immunosorbent assay (ELISA) kits for leptin (cat. no. ml-1015883), adiponectin (cat. no. ml-42683), and proinflammatory cytokines, including TNF-α (cat. no. ml-1015901), IL-1β (cat. no. ml-67771), and IL-6 (cat. no. ml-67768), were used to determine the levels of leptin, adiponectin, TNF-α, IL-1β, and IL-6 in EAT, following the manufacturer’s instructions (Shanghai MLBIO Biotechnology Co., Ltd., Shanghai, China).
RNA extraction from tissues and quantification by real-time qRT-PCR
To measure the differences in relative mRNA expression, we used qRT-PCR. The total RNA was extracted from the frozen EAT using TRIzol reagent (CoWin Biosciences (CWBIO), MA, USA) according to the manufacturer’s protocols, and the quality of total RNA was evaluated using a Nano-Drop One spectrophotometer (Thermo Scientific, Wilmington, DE, USA). Then, the cDNA was reverse transcribed from the total RNA using the HiFiScript gDNA Removal cDNA Synthesis Kit (Jiangsu Cowin Biotech Co., Ltd., Changping, Beijing, China). qRT-PCR was performed using SYBR PRIME qPCR Kits (Fast HS) on a CFX thermocycler system (Bio-Rad, Hercules, CA, USA). PCR amplification was performed as follows: 95°C for 5 min and 40 cycles of amplification at 94°C for 20s, 94°C for 10s, 50–60°C for 10s, and 72°C for 10s. Relative quantification was calculated using the 2−ΔΔCT method, and GAPDH, a housekeeping gene, was utilized to normalize the level of the target gene expression. The primer sequences used in this study are shown in Table 2.
Table 2
The primer sequences used for qPCR.
Histological analysis
The liver and EAT, freshly isolated from each of the five mice groups, were quickly fixed in 4% paraformaldehyde for 24 h at room temperature. The tissues were subsequently coated in paraffin and sliced into 5 μm thick sections. After staining slices with hematoxylin and eosin (H&E), histopathological alterations were examined using a light microscope (Olympus, Tokyo, Japan). The EAT number and size were measured by the software Image J (NIH Image, Bethesda, Rockville, MD, USA).
Statistical analysis
All data were obtained from at least three replications and presented as a means ± standard deviation (SD). Statistical analysis was performed using Statistix Analytical Software version 9.0 (Analytical Software, Tallahassee, FL, USA). The variances among the collected data were assessed by one-way ANOVA using the Tukey’s test, and differences at p<0.05 were considered statistically significant. The figures were designed by OriginPro 2021 software (OriginLab, Co., Northampton, MA, USA).
RESULTS AND DISCUSSION
Fatty acid composition
The fatty acid (FA) composition of the seed oils tested is shown in Table 3. Eleven FAs were detected in cold-pressed PSO, SFO, and TSO, of which six were SFAs, three were MUFAs, and the last two were PUFAs. Among six SFAs (myristic, palmitic, stearic, arachidic, behenic, and lignoceric acids), two were dominant: palmitic acid, which ranged from 5.02% in SFO to 8.64% in TSO, and stearic acid, which varied from 2.04% in TSO to 5.52% in SFO. Although there were minor variances in SFA content among the oils studied, SFO had the highest total SFA content (12.01%). Three MUFAs (palmitoleic, oleic, and gadoleic acids) were identified in all samples, but only one was dominant: oleic acid, which ranged from 15.62% in PSO to 78.33% in TSO. TSO had the highest MUFA content, which was substantially different from the other oils (p<0.05).
Table 3
Fatty acid composition of sunflower, perilla, and tea seed oils (% total fatty acids).
[i] Data are presented as mean ± standard deviation (n=3). Different letters in the same row indicate significant differences at p<0.05, according to Tukey’s test. FA, fatty acids; SFO, sunflower oil; PSO, perilla seed oil; TSO, tea seed oil; SFAs, saturated fatty acids; MUFAs, monounsaturated fatty acids; PUFAs, polyunsaturated fatty acids; USFAs, unsaturated fatty acids.
Among PUFAs, C18:2n6 and C18:3n3 contents varied from 8.50% and 0.11% to 50.66% and 61.58%, respectively. Linoleic acid was the most abundant FA in SFO, followed by PSO and TSO, while only PSO had a high content of α-linolenic acid. There were significant differences (p<0.05) in PUFA content among all tested oil samples, with PSO (74.24%) having the greatest content, followed by SFO (51.10%) and TSO (9.05%). The SFA:USFA ratio was 1:7, 1:8, and 1:8 in SFO, PSO, and TSO, respectively, suggesting all three examined oils have a low ratio of SFA:USFA. Interestingly, several previous studies have established that the SFA:USFA ratio is a significant health predictor, and diets high in MUFAs with a low SFA to USFA ratio may alleviate obesity [Liao et al., 2010; Yang et al., 2017].
Effects of PSO, SFO, and TSO supplementation on body weight and food intake in obese mice
Body weight is considered one of the most axiomatic indicators to judge obesity cases in mice. Here, during the experiment, the body weight growth of the HFD group increased faster than in the ND, PSO, SFO, and TSO groups (Figure 1B). The PSO and TSO groups had lower body weights than those in the HFD group after 8 weeks of oil supplementation, but there were no significant differences (p≥0.05) in body weight gain among the SFO and HFD groups, indicating that PSO and TSO supplementation could effectively prevent the body weight gain induced by the HFD (Figure 1C). In contrast, there were no significant differences (p≥0.05) between all the dietary oil and HFD groups in terms of food consumption (Figure 1D) and calorie intake (Figure 1E). Additionally, the HFD group’s food efficiency ratio (FER) was higher than that of the ND group (p<0.05), while the FER was significantly decreased after 8 weeks of supplementation with dietary oils, particularly in the PSO and TSO groups (Figure 1F).
Moreover, HFD feeding increased the relative weight of the liver, while dietary oil supplementation reversed this trend (Table 4). However, there were no significant differences (p≥0.05) in the relative weight of the kidney, lung, and spleen among the five mice groups. In contrast, compared to the HFD group, PSO, SFO, and TSO administration significantly reduced the proportion of EAT and PAT relative to the body weight (p<0.05).
Table 4
The relative tissue weights in mice of ND, HFD, PSO, SFO, and TSO groups (g/100 g body weight).
[i] Data are presented as mean ± standard deviation (n=8 for each group). Different letters in the same row indicate significant differences at p<0.05, according to Tukey’s test. ND, mice fed a normal diet; HFD, mice fed a high-fat diet; PSO, SFO, and TSO, mice fed a high-fat diet supplemented with PSO, SFO, and TSO at 2 g/kg body weight per day, respectively.
To sum up, dietary oil supplementation reduced body and organ weight gain but had no impact on food and calorie intake compared to the HFD group. In agreement with our results, α-linolenic acid-rich kiwifruit seed oil administered over 12 weeks decreased body weight gain without affecting food intake [Qu et al., 2019b]. Also, black raspberry seed oil, which is high in α-linolenic acid, reduced body weight but had no impact on food intake when administered for 10 weeks in db/db mice [Lee et al., 2016]. Cui et al. [2017] revealed that fish oil ingestion for 12 weeks caused body weight reduction in HFD-induced obese mice. In our study, there were no significant differences in food consumption among the five groups; therefore, the effect of dietary oil supplementation on body weight was not associated with the decrease in food intake.
Effects of PSO, SFO, and TSO supplementation on serum lipid profile levels
Obesity is strongly linked to changes in serum biochemistry. As a result, we used commercial kits to examine the effect of oil supplementation on serum lipid profile levels. As shown in Figure 2A, TG concentrations in the serum of mice of ND, HFD, PSO, SFO, and TSO groups were 1.60, 2.59, 2.13, 2.21, and 2.06 mmol/L, respectively, with the HFD group having a substantially higher TG concentration than the other groups (p<0.05). After 8 weeks of oils supplementation, the levels of TC were significantly lower (p<0.05) in the PSO, SFO, and TSO groups, by 25.70%, 15.36%, and 22.30%, respectively, compared with the HFD group (Figure 2B). However, the results of HDLC showed no significant differences (p≥0.05) in the HDL-C levels among the HFD and all other treatment groups, with the highest levels being observed in the ND group (Figure 2C). Notably, the high HDL-C level in the HFD group may represent an adaptive response to the increased demand for lipid transport [Wolf & Phil, 1996]. Furthermore, compared with the HFD group, the serum LDL-C levels were significantly reduced by 44.62%, 32.31%, and 51.79% in the PSO, SFO, and TSO groups, respectively (Figure 2D). As shown in Figure 2E, the dietary oil intervention reduced serum vLDL-C levels in TSO group; however, there were no significant differences between the HFD, PSO, and SFO groups. Moreover, compared to the HFD group, the PSO, SFO, and TSO groups exhibited a reduction in atherogenic index values of 42.5%, 15%, and 35.4%, respectively (Figure 2F).
Figure 2
Perilla seed oil (PSO), sunflower oil (SFO), and tea seed oil (TSO) supplementation reduced the serum lipid levels and atherogenic index in HFD-induced obese mice. (A) Triglycerides, TG; (B) Total cholesterol, TC; (C) High-density lipoprotein cholesterol, HDL-C; (D) Low-density lipoprotein cholesterol, LDL-C; (E) Very low-density lipoprotein cholesterol, vLDL-C; (F) Atherogenic index. ND, mice fed a normal diet; HFD, mice fed a high-fat diet; PSO, SFO, and TSO, mice fed a high-fat diet supplemented with PSO, SFO, and TSO at 2 g/kg body weight per day, respectively. Data are presented as mean ± standard deviation (n=8). Means with different letters (a–c) were considered significantly different at p<0.05, according to Tukey’s test.
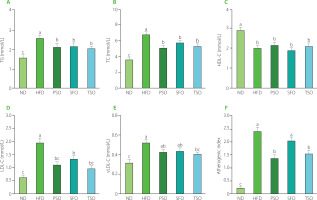
Our study revealed that long-term intake of PSO, SFO, and TSO reduced serum lipid profile levels and atherogenic index values, which are risk factors for CVD. These findings are consistent with the report by Lian et al. [2020] who showed that replacing SFAs with USFAs in the Western diet improved the blood lipid profile, increased USFA levels in plasma, and reduced the risk of atherosclerosis in mice. Consumption of fish oil caused significantly lower body weight and blood lipid concentrations in HFD-induced obese mice [Pradhan et al., 2020]. In a similar study, oral treatment of camellia oil considerably reduced fat deposits, serum TC, TG, LDL-C, and the atherogenic index, as well as significantly elevated HDL-C concentration in HFD-induced obese mice [Huang et al., 2021]. Our findings showed that PSO, SFO, and TSO consumption could remarkably improve lipid metabolism, alleviate dyslipidemia, and reduce the risk of atherosclerosis development in HFD-fed mice.
Effects of PSO, SFO, and TSO supplementation on fat tissue histopathology
The EAT, a metabolically active and anatomically distinct abdominal fat depot, is commonly employed to investigate adipose tissue in rodent studies [Wang et al., 2018]. As shown in Figure 3A, histopathology examination (H&E staining) of EAT revealed that HFD feeding encouraged adipocyte hypertrophy and that the HFD group had lower adipocyte numbers and larger adipose cell sizes than the ND group (Figures 3B and C). On the contrary, oral supplementation with dietary oils alleviated these effects, where the adipocytes of EAT in all treatment groups were small and closely organized with morphological integrity, particularly in PSO and TSO, suggesting that the studied oils have the potential to attenuate fat accumulation in mice caused by a HFD. Our results agreed with the previous studies, which showed that perilla oil supplementation reduced the adipocyte size and ameliorated the percentage of fat tissue in diabetic KK-Ay mice fed with HFD [Wang et al., 2018]. Another study indicated that consuming kiwifruit seed oil for 12 weeks reduced fat tissue weight compared with the HFD group, and this finding was corroborated by fat tissue histological examination in obese mice [Qu et al., 2019b]. These results indicate that supplementation with PSO, SFO, and TSO could reduce fat accumulation in adipose tissue.
Figure 3
Perilla seed oil (PSO), sunflower oil (SFO), and tea seed oil (TSO) supplementation reduced adipocyte hypertrophy and increased adipocyte numbers in the histopathological examination of epididymal adipose tissue. (A) Histological analysis of epididymal adipose tissue (hematoxylin and eosin staining, ×100 magnification); (B) Adipocyte numbers; (C) Adipocyte size. ND, mice fed a normal diet; HFD, mice fed a high-fat diet; PSO, SFO, and TSO, mice fed a high-fat diet supplemented with PSO, SFO, and TSO at 2 g/kg body weight per day, respectively. Data are presented as mean ± standard deviation (n=6–8). Means with different letters (a–d) were considered significantly different at p<0.05, according to Tukey’s test.
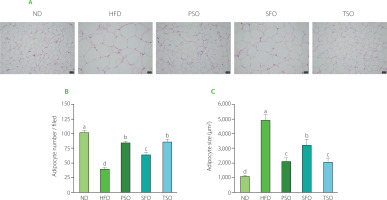
Effects of PSO, SFO, and TSO supplementation on liver function indicators and hepatic steatosis in obese mice
The liver is widely known for its role in detoxification and metabolic regulation. Therefore, it is critical to preserve the normal structure of the liver. To investigate the influence of oil supplementation on liver function, we measured the activity of serum AST and ALT, which are liver function representative markers that reflect the degree of liver injury [Chang et al., 2019]. As shown in Figures 4A and B, the ALT and AST levels were the lowest in the ND group mice. On the contrary, the HFD group mice had the highest levels, indicating liver injury. Also, ALT and AST levels were significantly reduced (p<0.05) in all dietary oil groups except the SFO group, where there were no significant differences in ALT levels compared to the HFD group. Furthermore, the effects of dietary oil supplementation on HFD-induced hepatic steatosis in mice by histological examination were investigated. H&E staining showed that the HFD-fed mice had more serious microvesicular steatosis and lipid droplet accumulation in hepatocytes than the ND-fed mice. At the same time, supplementation with dietary oils alleviated these adverse effects (Figure 4C), suggesting that the examined oils have a robust protective effect against HFD-induced liver injury. Our findings are consistent with prior observations in other rodent models that USFA oils have a defensive impact in HFD-induced obese mice [Su et al., 2016]. Our results allow us to conclude that PSO, SFO, and TSO supplementation could suppress HFD-induced obesity and regulate lipid metabolism by enhancing liver function and its structural integrity.
Figure 4
Perilla seed oil (PSO), sunflower oil (SFO), and tea seed oil (TSO) supplementation improved liver function indicators and alleviated liver steatosis. (A) Alanine aminotransferase (ALT) activity; (B) Aspartate aminotransferase (AST) activity; (C) Histological analysis of liver tissue (hematoxylin and eosin staining, ×100 magnification). ND, mice fed a normal diet; HFD, mice fed a high-fat diet; PSO, SFO, and TSO, mice fed a high-fat diet supplemented with PSO, SFO, and TSO at 2 g/kg body weight per day, respectively. Data are presented as mean ± standard deviation (n=6–8). Means with different letters (a–d) were considered significantly different at p<0.05, according to Tukey’s test.
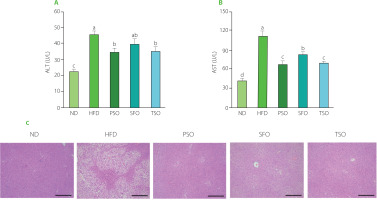
Effects of PSO, SFO, and TSO supplementation on serum glucose, insulin, and FFA levels
Next, commercial kits were used to investigate the effects of dietary oil supplementation on serum concentrations of insulin, glucose, and FFA in HFD-induced obese mice. Consumption of HFD generated a substantial rise in blood glucose concentration (10.57 mmol/L), which was much higher than in the ND group (5.82 mmol/L, p<0.05). In contrast, compared to the HFD group, supplementation with dietary oils markedly reduced the fasting serum glucose concentrations by 22.99%, 14.66%, and 17.88% in the PSO, SFO, and TSO, respectively (Figure 5A). In addition, the serum insulin level was remarkably higher in the HFD group compared to the ND group. After 8 weeks of dietary oil intervention, there were significant differences in insulin concentrations across all treatment groups except the SFO group (p<0.05, Figure 5B). Insulin resistance is characterized as an organ’s inability to respond to insulin. The HOMA-IR and HOMA-IS indices are common indicators used to estimate insulin resistance and sensitivity in diabetic patients. In our study, dietary oil supplementation for 8 weeks notably improved the HOMA-IR index; however, there were no significant differences in the HOMA-IS index among the SFO, TSO, and HFD groups (Figures 5C and D). Previous studies have found that supplementation with kiwifruit seed oil, which is also high in USFAs, reduced insulin and glucose levels in the blood and improved the indicator of insulin resistance in mice with HFD-induced obesity [Qu et al., 2019a, b].
Figure 5
Perilla seed oil (PSO), sunflower oil (SFO), and tea seed oil (TSO) supplementation alleviated diet-induced hyperglycemia, improved insulin resistance, and reduced serum free fatty acid (FFA) levels. (A) Fasting glucose level; (B) Insulin level; (C) Insulin resistance (HOMA-IR) index; (D) Insulin sensitivity (HOMA-IS) index; (E) FFA level. ND, mice fed a normal diet; HFD, mice fed a high-fat diet; PSO, SFO, and TSO, mice fed a high-fat diet supplemented with PSO, SFO, and TSO at 2 g/kg body weight per day, respectively. Data are presented as mean ± standard deviation (n=8). Means with different letters (a–d) were considered significantly different at p<0.05, according to Tukey’s test.
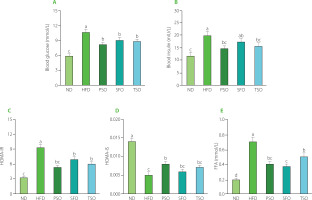
The liver is an important organ in lipid metabolic pathways because it takes up FFAs from the blood as well as produces, transports, and stores lipid metabolites. It is established that obesity, insulin resistance, type 2 diabetes, dyslipidemia, CVD, and atherosclerosis have all been associated with high levels of FFAs [Henderson, 2021]. Furthermore, a potential association between inflammation and serum FFAs has been suggested in both animal and human studies. A high level of FFAs in the bloodstream is closely associated with the onset of inflammation, noted by the increase in the secretion of IL-6 and TNF-α in adipose tissues [Neeland et al., 2018]. Additionally, inflammatory cytokines promote lipolysis and raise FFA levels, contributing to endothelial dysfunction and increasing the risk of atherosclerosis [Ormseth et al., 2013]. As shown in Figure 5E, the serum FFA level in the HFD group (0.71 mmol/L) increased by 255% compared to the level in the ND group (0.20 mmol/L). After 8 weeks of dietary oil supplementation, the FFA levels in the serum were significantly reduced by 42%, 46%, and 28% in the PSO, SFO, and TSO groups, respectively, but still higher than those in the ND group. Our findings indicate that consumption of PSO, SFO, and TS could alleviate hyperglycemia, insulin resistance, atherosclerosis, and inflammation by preventing lipolysis and reducing serum FFA levels in HFD-fed mice in a FA composition-dependent way.
Effects of PSO, SFO, and TSO supplementation on secretion and mRNA expression of inflammatory cytokines in EAT
Generally, adipose tissue produces a variety of factors with biological activity, including inflammatory hormones (leptin and adiponectin), pro-inflammatory cytokines (TNF-α, IL-1β, and IL-6), and anti-inflammatory cytokines (IL-10) [Hummasti & Hotamisligil, 2010]. Furthermore, in obese individuals, adipocyte hypertrophy promotes an increase in the release of inflammatory cytokines, which induces adipose tissue metabolic inflammation and progressively leads to chronic low-grade inflammation [Hummasti & Hotamisligil, 2010; Oh & Olefsky, 2012]. Therefore, obesity is commonly related to chronic low-grade inflammation. Thus, secretion and mRNA expression levels of inflammation-related genes in the EAT were measured to clarify whether consuming vegetable oils can alleviate the inflammation caused by a HFD.
It is well known that leptin, an adipose-derived hormone, regulates body weight by affecting food consumption and energy expenditure [Williams et al., 2009], and its levels increase in patients with obesity and decrease in individuals with weight loss. Obesity stimulates the secretion and production of proinflammatory cytokines such as TNF-α, IL-6, and IL-1β in adipose tissue [Trayhurn & Wood, 2004]. TNF-α has been linked to the development of obesity, insulin resistance, and type 2 diabetes [Golshani et al., 2015]. Furthermore, IL-6 has a potent pro-inflammatory effect, which can increase TNF-α levels [Park et al., 2005]. On the other hand, adiponectin also modulates lipid and glucose metabolism, protects against chronic inflammation, and improves insulin sensitivity [Pajvani et al., 2004]. Its lower levels are associated with obesity and related diseases such as type 2 diabetes, non-alcoholic fatty liver disease, and insulin resistance [Ebrahimi et al., 2022]. As shown in Figure 6A, HFD feeding led to a 3.45-fold rise in EAT levels of leptin in the HFD group compared with the ND group (p<0.05). At the same time, dietary oil supplementation lowered leptin levels by 40.31%, 37.19%, and 35.54% in the PSO, SFO, and TSO groups, respectively, compared to the HFD group. Moreover, EAT levels of pro-inflammatory cytokines (TNF-α, IL-6, and IL-1β) were considerably higher in the HFD group when compared to the ND group; however, following 8 weeks of dietary oil supplementation, the concentrations decreased in the PSO, SFO, and TSO groups (Figures 6B, C, and D). On the contrary, supplementation with dietary oils significantly elevated the EAT levels of anti-inflammatory adipokine adiponectin by 20.20%, 11.74%, and 10.64% in the PSO, SFO, and TSO groups, respectively, when compared with the HFD group (p<0.05, Figure 6E). Furthermore, the same trends were approximately found in mRNA expression levels (Figure 6F). Our results were consistent with the previous studies, which showed that the HFD supplemented with fish oil lowered leptin levels while increasing adiponectin levels in adipose tissue [Sundaram et al., 2016]. Thomas et al. [2020a] found that perilla oil administration reduced pro-inflammatory cytokines in serum and colon, and the effects were comparable to those obtained from fish oil in HFD-induced inflammation in mice. Wu et al. [2020] observed that camellia oil intake significantly reduced pro-inflammatory cytokine levels (TNF-α, IL-1β, and IL-6) in rats with acetic acid-induced colitis. Another study also demonstrated that kiwifruit seed oil supplementation decreased the mRNA expression of pro-inflammatory cytokines (TNF-α, IL-6, and IL-1β) in a dose-dependent way, compared to the HFD group [Qu et al., 2019b]. Furthermore, fish oil addition to a HFD significantly raised adiponectin levels and lowered leptin levels in serum and EAT mRNA expression, according to a study published by Monk et al. [2019]. Our findings show that USFA in the examined oils could relieve low-grade adipose tissue inflammation in obese mice.
Figure 6
Effects of perilla seed oil (PSO), sunflower oil (SFO), and tea seed oil (TSO) supplementation on the secretion and mRNA expression levels of inflammatory cytokines of epididymal adipose tissue in HFD-induced obese mice. (A) Leptin; (B) tumor necrosis factor-alpha, (IL-6), and TNF-α; (C) interleukin-6, IL-6; (D) interleukin-1beta, IL-1β; (E) Adiponectin; (F) Relative mRNA expression. ND, mice fed a normal diet; HFD, mice fed a high-fat diet; PSO, SFO, and TSO, mice fed a high-fat diet supplemented with PSO, SFO, and TSO at 2 g/kg body weight per day, respectively. Data are presented as mean ± standard deviation (n=8). Means with different letters (a–d) were considered significantly different at p<0.05, according to Tukey’s test.
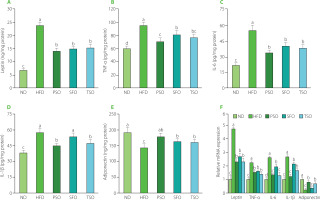
Effects of PSO, SFO, and TSO supplementation on mRNA expressions of lipid metabolism-related genes of EAT in mice
Next, the effects of oils supplementation on specific essential lipid metabolism-related genes were also examined to clarify further the mechanism and possible effect of vegetable oils on lipid metabolism dysfunction. Several regulatory factors, including the nuclear receptor family of peroxisome proliferator-activated receptors (PPARs), have been found to play a vital regulatory role in energy homeostasis and metabolic functions [Wagner & Wagner, 2020]. Numerous PPAR subtypes have been identified, among which are PPAR-α and PPAR-γ. PPAR-α controls the expression of several lipid metabolismrelated genes and is a critical target for treating lipid metabolism disorders [Kersten & Stienstra, 2017]. PPAR-γ regulates the primary genes involved in the production and lipid metabolism, as well as the transport and accumulation of FAs [Janani & Ranjitha Kumari, 2015]. In addition, FAS, the major adipogenesis enzyme involved in lipid synthesis, is a sign of the obesity therapeutic impact [Noratto et al., 2016]. As shown in Figure 7A, the mRNA expression level of PPAR-α was up-regulated in the three oil-supplemented groups compared to the HFD group (p<0.05), and there were no significant differences (p≥0.05) among the PSO and TSO groups. Compared to the ND group, the expression levels of PPAR-γ (Figure 7B) and FAS (Figure 7C) were significantly increased, but after 8 weeks of dietary oils intervention, the expression levels of those genes were substantially lower than those in the HFD group (p<0.05). Supplementation with dietary oils significantly reduced FAS expression, which indicated the downregulation of FA synthesis. Importantly, dietary oils also suppressed lipid accumulation by inactivating the expression of PPAR-γ [Jiang et al., 2014; Zhang et al., 2020]. Altogether, these findings indicate that PSO, SFO, and TSO could exert anti-obesity effects by downregulating lipid synthesis and accumulation.
Figure 7
Effects of perilla seed oil (PSO), sunflower oil (SFO), and tea seed oil (TSO) supplementation on the mRNA expression levels of lipid metabolism-related genes in epididymal adipose tissue. (A) peroxisome proliferator-activated receptor-alpha, PPAR-α; (B) peroxisome proliferator-activated receptor-gamma, PPAR-γ; (C) fatty acid synthase, FAS; (D) carnitine palmitoyl transferase 1a, CPT1a; (E) carnitine palmitoyltransferase 1b, CPT1b; (F) uncoupling protein 1, UCP1. ND, mice fed a normal diet; HFD, mice fed a high-fat diet; PSO, SFO, and TSO, mice fed a high-fat diet supplemented with PSO, SFO, and TSO at 2 g/kg body weight per day, respectively. Data are presented as mean ± standard deviation (n=8). Means with different letters (a–d) were considered significantly different at p<0.05, according to Tukey’s test.
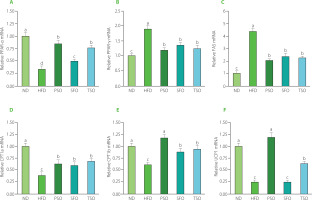
Moreover, we also measured mRNA expression levels of both CPT1a and CPT1b, which are enzymes controlling the rate in the pathway of FA β-oxidation [Kliewer et al., 2001]. Consumption of HFD resulted in downregulated mRNA expression levels of both CPT1a and CPT1b compared to the ND group, while the levels were significantly increased in all dietary oil groups (p<0.05, Figures 7D and E). Next, we measured the mRNA expression levels of thermogenesis-linked genes, such as UCP1, which is considered a morphological and functional sign of thermogenic adipocyte production [Zhang et al., 2016]. As shown in Figure 6F, the expression levels of the UCP1 gene were significantly up-regulated after 8 weeks of dietary oil intake in both the PSO and TSO groups compared to the levels in the HFD group, but there were no significant differences between the SFO and HFD groups. Notably, it has been reported that activating UCP1-expressing adipocytes has effective potential as an antidiabetic and anti-obesity agent by increasing energy consumption and lowering adiposity in mice [Bond & Ntambi, 2018]. Our findings were consistent with the previous studies, which found that up-regulation of FA oxidation by PPAR-α was associated with a decrease in fat accumulation in HFD-induced obesity [Bremer, 2001; Hirako et al., 2010]. Another study revealed that HFD supplemented with perilla, olive, and safflower oils exerted anti-obesity effects by reducing expression levels of genes associated with lipid synthesis (PPAR-γ and FAS) and increased expression levels of genes related to FA β-oxidation (PPAR-α) in both liver and adipose tissue in mice with obesity and colon inflammation triggered by HFD [Thomas et al., 2020a]. A similar study also showed that consumption of kiwifruit seed oil had anti-obesity effects by increasing mRNA expression levels of FA β-oxidation-related genes (PPAR-α, CPT1a, and CPT1b) and energy metabolism and thermogenesis (UCP2) while decreasing mRNA expression levels of lipid synthesis-related genes (PPAR-γ and FAS) in obese mice induced by HFD [Qu et al., 2019a, b]. Our study found that PSO, SFO, and TSO supplementation suppressed PPAR-γ and FAS expressions and enhanced PPAR-α, CPT1a, CPT1b, and UCP1 expressions to different degrees, revealing that dietary oil supplementation had a strong inhibitory effect on lipid metabolism disorders in HFD-fed mice.
The FA composition analysis showed that PSO, SFO, and TSO had an incredibly high content of USFAs and a low SFA:USFA ratio (Table 3), indicating that the anti-obesity properties of these oils are probably due to the high content of USFA and the low SFA:USFA ratio, especially in PSO and TSO. Previous studies have shown that a low SFA:USFA ratio is beneficial for health [Li et al., 2015; Thomas et al., 2020a]. Interestingly, although the three oils studied showed similar anti-obesity effects in HFD-fed mice, there were some subtle differences in the metabolic effects. Notably, dietary oils vary in FA composition and the presence of other active minor compounds, such as tocopherols, carotenoids, etc. These variations are believed to lead to various biochemical pathways and, thus, different metabolic impacts [Altberg et al., 2020]. For instance, the molecular technicalities that underlie the effects of PSO in preventing lipid accumulation, inhibiting hyperglycemia, and improving lipid metabolism and chronic inflammation are likely related to the bioconversion of α-linolenic acid to eicosapentaenoic acid (C20:5n3) and docosahexaenoic acid (C22:6n3) [González-Mañán et al., 2012]. These findings highlight the necessity of replacing SFAs with USFAs and the potential applications for by-products of these dietary oils in the context of a balanced and healthy dietary pattern. However, the mechanisms through which PSO, SFO, and TSO ameliorate inflammation and lipid metabolism in HFD-induced obese mice must be further investigated. It has been shown that the gut microbiota is a critical factor in body health regulation and might be a target for treating inflammation, obesity and other chronic diseases [Scheithauer et al., 2020]. Therefore, an exciting study will be conducted to explore whether PSO, SFO, and TSO supplementation can ameliorate obesity by modulating the gut microbiota composition compared with one of the most commonly used anti-obesity drugs.
CONCLUSIONS
In this study, we investigated the effect of oral supplementation with PSO, SFO, and TSO, oils with different fatty acid compositions, on HFD-induced obesity in KM mice. The results of the animal study demonstrated that oils supplementation could reduce body weight and tissue weight but not food intake in HFD-induced obese mice. Furthermore, PSO, SFO, and TSO supplementation alleviated hyperlipidemia, hyperglycemia, and hepatic steatosis induced by HFD, reduced serum FFA levels, and improved insulin resistance, liver function, and the atherogenic index. Additionally, the results showed that dietary oil supplementation suppressed HFD-induced adipose tissue inflammation in mice by reducing both secretion and mRNA expression levels of pro-inflammatory cytokines and increasing secretion and mRNA expression levels of anti-inflammatory adipokines. Likewise, the qPCR assay of lipid metabolism-related genes showed that dietary oil supplementation improved lipid metabolism by increasing FA β-oxidation and thermogenesis and suppressing lipid synthesis in EAT. Taken together, PSO, SFO, and TSO supplements could have potential anti-obesity implications by suppressing inflammation and improving lipid metabolism in HFD-induced obese mice.