ABBREVIATIONS
ABTS – 2,2′-Azino-bis(3-ethylbenzthiazoline)-6-sulfonic acid; ABTS•+ – 2,2′-Azino-bis(3-ethylbenzthiazoline)-6-sulfonic acid radical cation; ACE – Angiotensin I-converting enzyme; DDW – Deionized distilled water; DPPH – 2,2-Diphenyl-1-picrylhydrazyl; EAA – Essential amino acid; EAI – Emulsifying activity index; ESI – Emulsion stability index; EW – Egg white powder; FC – Foaming capacity; FS – Foaming stability; HAA – Hydrophobic amino acid; HPAA – Hydrophilic amino acid; OHC – Oil holding capacity; PUFAs – Polyunsaturated fatty acids; SP – Soy protein concentrate; TL – Tuna liver; TLP – Tuna liver protein powder; TLP 2.5 – Tuna liver protein powder from solubilization at pH 2.5; TLP 11.5 – Tuna liver protein powder from solubilization at pH 11.5; WHC – Water holding capacity.
INTRODUCTION
Skipjack tuna (Katsuwonus pelamis) is a major fish species used by the canned tuna industry and other processed tuna products. In Thailand, the annual production of canned tuna has remarkably increased and reached approximately 445,000 tons, and the value exceeded 1,677 million US dollars in 2021 [Department of Fisheries, 2022]. Following the annual growth of the canned tuna industry, the discarding rate of fish processing by-products has also remarkably increased.
Tuna liver (TL) is an abundant solid by-product which has long been underutilized. Commonly, most TL is used in the production of low-market value products, such as fish feed meal, or left as waste, which creates huge economic and environmental concerns [Shen et al., 2022]. However, TL is nutritionally rich in protein, the content of which reaches 18 g/100 g [Kang et al., 2007]. The most abundant essential amino acids of proteins include leucine, lysine, and valine and the prominent non-essential amino acids are aspartic acid, glutamic acid, and alanine [Kang et al., 2007; Shen et al., 2022]. Recently, there has been a great interest in increasing the utilization of TL as a potential source of protein-based food for functional ingredients [Shen et al., 2022].
The pH-shift process, mainly acidic or alkaline solubilization followed by the isoelectric precipitation of proteins, is a potentially applicable technique that efficiently recovers functional and nutritional proteins from fish processing by-products that otherwise would be discarded from direct human consumption [Chomnawang & Yongsawatdigul, 2013; Kang et al., 2018; Kristinsson et al., 2013]. The recovered protein isolate can be directly dried into a shelf-stable fish protein powder for further utilization. To date, the pH shift process has been remarkably applied to recover protein from different varieties of fish processing by-products, such as salmon, cod, and herring, for subsequent development of alternative and functional food ingredients [Abdollahi & Undeland, 2019]. To the best of our knowledge, limited reports are available on the functional properties and bioactivities of protein powder produced from skipjack TL using the pH shift process.
From the above mentioned, by-products pose a significant problem for the canned tuna industry because it leads to environmental impact. However, tuna liver is characterized by a high protein content and can be used as a protein source in foods. The utilization of TL for human consumption could be an innovative strategy for eco-friendliness between industry and food sustainability for consumers. Therefore, this study aimed to evaluate the nutritional and functional properties as well as antioxidant and angiotensin I-converting enzyme (ACE) inhibitory activities of protein powder prepared from skipjack TL using the pH shift process to enable future development of the use of TL protein as a valuable food.
MATERIALS AND METHODS
Raw materials and sample preparation
Frozen livers of skipjack tuna (Katsuwonus pelamis; body length of 43–53 cm and body weight of 4.5–5.5 kg) were kindly donated by a canned tuna processing plant (Samut Sakhon Province, Thailand), and transported in an icebox at approximately 4°C to the laboratory of the Department of Fishery Products, Kasetsart University, Bangkok within 2 h. After arrival, the tuna livers (TL) were immediately stored at –20°C until use and they were thawed for 24 h at 4°C prior to experiments. The liver was then cut into small pieces and ground using a laboratory blender (Waring Commercial, Torrington, CT, USA). A portion of ground liver was directly used as a raw material for protein isolation. The remaining portions of the ground liver were freeze-dried using freeze dryer (Coolsafe 95–15, Labogene, Lillerød, Denmark), ground, and kept as lyophilized powder in a sealed polyethylene bag at –20°C until further use in the analysis. The ground TL powder was marked as the control.
Determination of tuna liver protein solubility
To determine the protein solubility of TL at different pHs, the method described by Li et al. [2017] was used with slight modifications of the sample-to-solvent ratio. A flowchart of TL protein solubilization is shown in Figure 1A. Initially, the ground TL (20 g) was homogenized with six volumes of cold deionized distilled water (DDW) using a homogenizer (Ultra-Turrax T25; IKA®, Staufen, Germany) at 10,000 rpm for 1 min. The homogenate was adjusted to an acidic or alkaline pH from 1.5 to 12.5 (1.0 pH intervals) by adding 1 M HCl or 1 M NaOH during constant automatic stirring. After incubation for 10 min, the sample was centrifuged at 10,000×g for 10 min at 4°C using a refrigerated centrifuge (Tomy Seiko Co. LTD., Tokyo, Japan). The middle layer of the supernatant was collected, and the protein concentration was quantified by the Lowry method using bovine serum albumin (Sigma-Aldrich, St. Louis, MO, USA) as a standard protein [Lowry et al., 1951]. The protein solubility was calculated and expressed as mg/mL, and a solubility curve (solubility vs. pH) was generated.
Preparation of the tuna liver protein powder using the pH shift processes
The protein was recovered from TL using the pH shift process according to the method described by Kristinsson et al. [2013] with some modifications in the pH of protein solubilization step. A flowchart of TLP preparation is shown in Figure 1. Briefly, the ground liver was suspended in DDW at a ratio of 1:6 (w/v) and then homogenized in an ice bath at 10,000 rpm for 1 min. The homogenate was adjusted to the acidic pH (2.5 and 3.5) or alkaline pH (10.5 and 11.5) by the drop-wise addition of 1 M HCl or 1 M NaOH with continuous stirring at 4°C until reaching the maximum solubility points as determined by the solubility profile. The mixtures were left for 10 min at 4°C and then centrifuged at 10,000×g for 10 min at 4°C to separate the lipids (upper layer) and insoluble residues (sediment). The middle layer with soluble proteins was collected and pH was adjusted to 5.5 using 1 M HCl or 1 M NaOH to accomplish the precipitation of the solubilized proteins. Thereafter, the precipitated protein was recovered by centrifugation at 10,000×g for 10 min at 4°C and additionally suspended in DDW through homogenization for 1 min. The pH of the homogenate was neutralized (pH 7.0) and the protein was finally collected via centrifugation at 10,000×g for 10 min at 4°C. The TL proteins prepared by the pH shift process (pH 2.5, 3.5, 10.5, and 11.5) were freeze-dried, ground, and marked as TLP 2.5, TLP 3.5, TLP 10.5, and TLP 11.5, respectively. The TLPs were stored in a sealed polyethylene bag at –20 °C until further use in the analysis.
Determination of extraction yield and protein recovery yield
The extraction yield of the TLP samples (TLP 2.5, TLP 3.5, TLP 10.5, and TLP 11.5) was calculated as a percentage according to the following Equation (1):
where, dry weight (g) of TLP and TL was used.The protein recovery yield of the TLP samples was calculated as a percentage according to the Equation (2) after determination of TLP and TL protein contents using the Kjeldahl method, specifically the conversion factor of 6.25 [AOAC, 2000].
Proximate composition analysis
The proximate composition (content of moisture, protein, lipid, and ash) of the TL powder and TLPs was analyzed according to the AOAC International [2000] following the analytical methods no. 950.46, 920.153, 960.39, and 928.08, respectively.
Color parameters analysis
The color attributes of the samples were determined using an UltraScan XE Hunter Lab tristimulus colorimeter (Hunter Assoc. Laboratory, Reston, VA, USA). Initially, the TL powder and TLPs were put in a transparent glass sample cup. The colorimeter was calibrated using a white standard plate and the lightness (L*), redness (a*), and yellowness (b*) were measured. Whiteness was calculated using the following Equation (3):
Visualization of appearance
The appearance of TL powder and TLPs was recorded using a digital camera (iPhone 11 Pro max; Apple, Cupertino, CA, USA).
Amino acid analysis
The amino acid profile analysis was conducted according to the method described by Jajic et al. [2013] with some modifications in the reagent for sample hydrolysis by excluding phenol. The TL powder and TLPs (0.5 g) were hydrolyzed with 5 mL of 6 M HCl using an oil bath (B-300, Buchi, Flawil, Switzerland) at 110°C for 24 h. The reaction mixture was finally diluted with water to 50 mL (HPLC grade) and then filtered through a 0.22-μm nylon membrane filter (Pall, Ann Arbor, MI, USA) prior to the amino acid analysis using high-performance liquid chromatography system (HPLC; Agilent 1200 infinity series LC system; Agilent Technologies, Palo Alto, CA, USA) equipped with a Poroshell-120 HPH-C18 column (4.6×100 mm, 2.7 µm particle size; Agilent Technologies). A 10-µL portion of the sample was injected at a flow rate of 1.0 mL/min. The column oven temperature was set at 40°C. Mobile phase A (pH 8.2) consisted of 10 mM disodium hydrogen phosphate (Na2HPO4), 10 mM sodium tetraborate (Na2B4O7×10H2O), and 0.5 mM sodium azide (NaN3). Mobile phase B consisted of acetonitrile, methanol, and water (45:45:10, v/v/v). All the reagents used for mobile phase preparation were of HPLC grade. The gradient conditions were set as follows: 2% B at 0–0.35 min, 2–57% B at 0.35–13.4 min, 57–100% B at 13.4–13.5 min, 100% B at 13.5–15.7 min, 100–2% B at 15.7–15.8 min, and 2% B at 15.8–18 min. The eluted amino acids were detected by monitoring at 230 nm for an excitation wavelength and at 450 nm for an emission wavelength using a fluorescence detector (FLD). Amino acids were identified and quantified based on the peak area integration using the peak area determined from a known amount of a mixed amino acid standard (0.2 mM solution; Agilent Technologies) for comparison. The data were expressed as g/100 g of protein.
Functional properties determination
Two commercial protein powders (soy protein concentrate, SP; and egg white, EW) were used as positive controls to estimate the solubility and functional properties of the TLPs from skipjack TL.
Protein solubility
The protein solubility of the TLPs was determined using the method described by Cha et al. [2020] with slight modifications in sample concentration. About 10 mg of freeze-dried sample was dispersed in 10 mL of distilled water, and the suspension was adjusted to pH values of 2, 3, 4, 5, 6, 7, 8, 9, 10, 11, and 12 using 0.1 M HCl or 1 M NaOH, under continuous stirring at room temperature. The mixture was stirred magnetically for 30 min and centrifuged at 10,000×g for 15 min at 4°C. The protein content in the supernatant and total protein content in the sample after solubilizing with 0.1 M NaOH were determined according to the method described by Lowry et al. [1951] using bovine serum albumin (BSA) as a protein standard. The relative protein solubility (%) of the TLPs was calculated using the following Equation (4):
Emulsifying properties
The emulsifying activity index (EAI) and emulsion stability index (ESI) of TLPs were evaluated following the method explained by Han et al. [2019] with some modifications of oil type and amount of emulsion sample. Initially, an emulsion was prepared by homogenizing the mixture of palm oil and protein solution (10 mg/mL) at a 1:3 (v/v) ratio at a speed of 10,000 rpm for 1 min. Then, a 50 µL aliquot of the emulsion at the bottom layer of the container at 0 and 10 min after homogenization was collected and transferred to another tube containing 0.1% sodium dodecyl sulfate solution (5 mL). Thereafter, the mixture was mixed thoroughly for 30 s using a vortex mixer. The absorbance of the resulting emulsion was measured at 500 nm using a UV-Vis spectrophotometer (Evolution 300, Thermo Scientific, Waltham, MA, USA). The EAI (m2/g) and ESI (min) values of the TLPs were calculated using the Equations (5) and (6), respectively:
where: dil is the dilution factor (100), A is the absorbance measured immediately after emulsion formation, C is the protein concentration (g/mL) before emulsion formation in the aqueous phase, and Φ is the oil volume fraction of the emulsion (0.25). where: A0 is the absorbance at 0 min, A10 is the absorbance at 10 min after homogenization, and t is the time between measurements (10 min).Foaming properties
The foaming capacity (FC) and foaming stability (FS) of the TLPs were analyzed following the method described by Cha et al. [2020]. To this end, 10 mL of sample solution (10 mg/mL) was homogenized at a speed of 10,000 rpm for 3 min and then the sample solution was immediately moved to a 50-mL cylinder. The sample was left undisturbed for 0 and 60 min. The FC (%) and FS (%) of each sample were calculated using the Equations (7) and (8), respectively:
where: V0 is the initial volume before whipping, VT is the total volume after whipping at 0 min, Vt is the total volume after whipping at 60 min, FT is the foam volume after whipping at 0 min Ft is the foam volume after whipping at 60 min.Water holding capacity (WHC)
The WHC of the TLPs was measured according to the method previously used by Han et al. [2019] with some modifications in centrifugation conditions. A 0.5-g portion of each sample was dispersed in 10 mL of distilled water and subsequently mixed for 60 s using a vortex mixer. After incubation at room temperature for 30 min, the solution was centrifuged at 6,000×g for 30 min and the volume of the resulting supernatant was measured. The difference between the initial volume of distilled water and the supernatant volume was calculated. The WHC was expressed as mL of absorbed water per g sample.
Oil holding capacity (OHC)
The OHC of the TLPs was determined following the method described by Han et al. [2019] with some modifications of oil type and the sample-to-oil ratio. Briefly, a 0.25 g portion of each sample was thoroughly mixed with 10 mL of palm oil for 60 s using a vortex mixer. After incubation at room temperature for 30 min, the sample was centrifuged at 6,000×g for 30 min and the difference between the supernatant and original volume of oil added to the sample was measured. The OHC was expressed as mL of oil per g sample.
Determination of antioxidant and antihypertensive activity
DPPH radical scavenging activity
The DPPH radical scavenging activity of TLPs was measured followed the method previously used by Yen & Hsieh [1995] with slight modifications in sample concentration. In brief, a reaction mixture of 1 mL of each sample solution (20 mg/mL) and 1 mL of 200 µM 2,2-diphenyl-1-picrylhydrazyl (DPPH) radical (Sigma-Aldrich) in ethanolic solution was mixed thoroughly using a vortex mixer. The mixture was left at room temperature for 30 min in the dark. The absorbance of the resulting mixtures was measured at 517 nm against a blank without DPPH radical using a UV-Vis spectrophotometer. Trolox (0–1.8 µM) was used for the standard curve preparation. The DPPH radical scavenging activity was expressed as µmol Trolox equivalent/mg protein.
ABTS radical cation scavenging activity
The 2,2′-azino-bis(3-ethylbenzothiazoline)-6-sulphonic acid (ABTS) radical cation scavenging activity was determined as described by Cha et al. [2020] with slight modifications in sample concentration. Briefly, the ABTS radical cation (ABTS•+) was generated by the reaction of ABTS stock solution (14.8 mM) and 5.2 mM potassium persulfate at room temperature for 12–16 h in the dark before use. The ABTS•+ solution was diluted with 200 mM phosphate buffer, pH 8.2 (1:1, v/v) to obtain an absorbance of 0.70±0.02 units using a UV-Vis spectrophotometer. About 0.5 mL of the protein sample solution (10 mg/mL) was mixed with 0.5 mL of DDW and 1 mL of ABTS•+ solution and the mixture was incubated at room temperature for 10 min in the dark. The absorbance was then measured at 734 nm. A standard curve of Trolox (0–200 µM) was plotted. The ABTS•+ scavenging activity was expressed as µmol Trolox equivalent/mg protein.
Ferrous-ion chelating activity
The ferrous-ion chelating activity was assessed using the method described by Torres-Fuentes et al. [2012] with slight modifications in sample concentration. To this end, 1 mL of the sample solution (0.1 g/mL) was mixed with 50 μL of 2 mM FeCl2 solution and 3.7 mL of distilled water. Then, 0.1 mL aliquot of 5 mM ferrozine solution was added and vortexed vigorously. After incubation for 20 min at room temperature, the absorbance of the reaction mixture was measured at 562 nm using a UV-Vis spectrophotometer. A control was evaluated by replacing the sample solution with 1 mL of distilled water. EDTA (0–100 µM) was used as a reference standard. The ferrous-ion chelating activity was expressed as µmol EDTA equivalent/mg protein.
Angiotensin-converting enzyme (ACE) inhibitory activity
The ACE-inhibitory activity was elucidated using the method previously used by Kasiwut et al. [2019] with modifications in sample concentration. A mixture of 100 μL of the protein sample solution (50 mg/mL) and 150 μL of 8.3 mM hippuryl-histidyl-leucine (HHL) solution was pre-incubated at 37°C for 10 min. The reaction was initiated by adding 50 μL of 25 mU/mL ACE solution (prepared in 50 mM sodium borate buffer containing 300 mM sodium chloride and adjusted to pH 8.3) and the sample was then incubated at 37°C for 30 min. The reaction was stopped by adding 250 μL of 1 M HCl and mixing well. The resulting hippuric acid was extracted with 1 mL of ethyl acetate. Thereafter, 800 μL of the upper layer was transferred into a test tube and vacuum dried at 105°C for 2 h. The hippuric acid was dissolved in 5 mL of distilled water, and the absorbance was measured immediately at 228 nm using a UV-Vis spectrophotometer. The percentage of ACE inhibitory activity of TLP was calculated as a percentage using Equation (9):
where: Acontrol is the absorbance readings of the buffer, Asample is the absorbance readings of the TLP solutions, and Ablank is the absorbance when the stop solution was added before the reaction occurred.Statistical analysis
All analyses were performed in triplicate. The results were expressed as mean ± standard deviations (n=3). The statistical analyses were conducted using SPSS version 26 (SPSS Inc., Chicago, IL, USA). The data sets were subjected to one-way analysis of variance (ANOVA), followed by Duncan’s multiple range post hoc test, and a significance level of p<0.05 was employed.
RESULTS AND DISCUSSION
Effects of pH on the protein solubility of tuna liver
To obtain a higher recovery of skipjack TLPs using the pH shift process, the solubility of proteins at various pH conditions (pH 1.5–12.5) was evaluated as shown in Figure 2. The protein solubility profile of the TL exhibited a rough V-shaped pattern. Protein solubility drastically increased when the pH was shifted to either side, ranging from pH 4.5 to 3.5 and pH 7.5 to 11.5. The maximum protein solubility was observed at pH 2.5 to 3.5 on the acidic side (0.46–0.49 mg/mL) and at pH 10.5 to 11.5 on the alkaline side (0.68–0.70 mg/mL), respectively. However, the minimum protein solubility was found at pH 5.5 (0.24 mg/mL) which may correspond to the isoelectric point of TLP due to the diminishment of electrostatic repulsions and the formation of large particles [Shen et al., 2022]. Similar findings were also reported in the protein solubility of fish muscle proteins such as tilapia [Chomnawang & Yongsawatdigul, 2013], rainbow trout [Lone et al., 2015], and yellowfin tuna liver [Shen et al., 2022]. In addition, it has been reported that the maximum solubility of chicken liver and goose liver proteins was observed at both extremely acidic and alkaline pH, ranging from pH 2.0 to 3.5 and 10.5 to 11.5, respectively, and the minimum solubility was observed at pH 5.0–5.5 [Li et al., 2017; Xiong et al., 2016]. The solubility of proteins has been increased in highly acidic or alkaline pH conditions due to an increase in the positive or negative charges of muscle proteins when the pH moved away from the isoelectric point which results in electrostatic repulsions of the protein molecules and the hydration of charged proteins [Xiong et al., 2016]. Based on the above results, the maximum solubility at pH 2.5 to 3.5 from the acidic side and pH 10.5 to 11.5 from the alkaline side were selected for the TL protein solubilizations, and pH 5.5 was chosen for the TL protein precipitations.
Extraction yield and protein recovery yield
The extraction yield of the TLPs under both acidic (pH 2.5 and 3.5) and alkaline (pH 10.5 and 11.5) pH conditions were determined as shown in Figure 3A. The yields of the TLP samples at pHs 2.5, 3.5, 10.5, and 11.5 were 13.85, 5.41, 39.61, and 48.82% (dry weight basis), respectively. This could be explained by the conformational changes of the proteins at extreme alkaline pHs causing the exposure of the buried groups in the protein structure which led to a higher yield [Abdollahi & Undeland, 2019]. Of note, the protein recovery yields of the TLPs were also evaluated. As shown in Figure 3B, the protein recovery yields in alkaline conditions were significantly (p<0.05) higher than those in acidic conditions. The maximum protein recovery yield was obtained from the alkaline pH shift at pH 11.5 (62.84%), whereas the recovery yield at the acidic pH shift was significantly (p<0.05) lower. This might be related to the increase in the electrostatic charge of the protein that consequently increased the protein solubility and yield. On the other hand, the lower protein recovery yield under acidic conditions might be caused by the trapping of solubilized protein in the sediment during the first centrifugation [Abdollahi & Undeland, 2019]. In addition, the higher protein recovery yields, as observed in the present study, were closely correlated to the solubility differences (p<0.05) of the TLPs under acidic or alkaline conditions (Figure 2). Even though the protein solubility at pH 3.5 was slightly higher than that at pH 2.5 (Figure 2), the protein recovery yield at pH 3.5 was the lowest among all treatments (8.74% dry weight basis; Figure 3B). This might be explained by the increase in the polarity of the protein at pHs below or above the isoelectric point which, in turn, raises the solubility of the proteins [Xiong et al., 2016]. A similar finding has been reported in yellowfin tuna liver where alkaline conditions resulted in a higher protein recovery yield than acidic conditions [Shen et al., 2022]. Different fish species and by-products, such as trout, silver carp, rohu, croaker, and anchovy, have shown a wide range of protein recovery yields ranging from 32–90% according to the pH shift process [Nolsøe & Undeland, 2009]. In addition, the yield of protein recovery was affected by many factors, such as the origin of raw material, centrifugal force, trapping of the solubilized proteins, protein amino acid composition, and the ratio between the raw materials and water [Marmon & Undeland, 2010]. Considering the above results, the optimal pHs to produce recovered proteins from TL using the pH shift process were pH 2.5 and pH 11.5.
Proximate composition
The proximate compositions of the TL powder (control) and TLPs (TLP 2.5 and TLP 11.5) are presented in Table 1. The moisture content of the TL powder, TLP 2.5, and TLP 11.5 was 9.74, 8.19, and 8.86 g/100 g, respectively. These results are important to consider as they strongly affect the protein powder properties [Pires et al., 2012]. Both TLP 2.5 and TLP 11.5 had a protein content of 68.09 and 55.25 g/100 g, which were greater (p<0.05) than the control (42.93 g/100 g). This result could be explained by the loss of connective tissue during centrifugation under the alkaline pH shift process [Kristinsson et al., 2006]. Therefore, the protein content was lower in TLP 11.5 than TLP 2.5. This result agreed well with the previous findings wherein acidic solubilization increased the protein content of pelagic fish and green crab compared to alkaline solubilization [Kang et al., 2018; Kristinsson et al., 2005]. The total lipid content changed from 14.98 g/100 g in the TL powder to 0.58 g/100 g in TLP 2.5 and 0.28 g/100 g in TLP 11.5 after the pH shift process due to the separation of lipids from proteins at very low or high pHs and a subsequent lipid migration into the processed water during centrifugation by the different solubility and density [Kristinsson et al., 2005; 2006]. The ash content of TLP 11.5 was significantly (p<0.05) higher than that of the control and TLP 2.5 (6.63, 4.68, and 2.30 g/100 g, respectively). This was probably due to the higher amount of NaOH and HCl used to adjust the pH at the alkaline conditions and during protein precipitation, resulting in a higher NaCl formation [Chomnawang & Yongsawatdigul, 2013]. Overall, the TLPs obtained following the pH shift process may have potential as an alternative source of protein with a low lipid content.
Table 1
Proximate composition, color parameters, and visual appearance of tuna liver powder (control) and tuna liver protein powders (TLPs) obtained following the pH shift process.
Color parameters and visual appearance
The color parameter differences among the TL powder and TLPs are shown in Table 1. The L* of TLP 2.5 (56.07) was significantly (p<0.05) higher than that of the TL powder and TLP 11.5 (40.05 and 45.39, respectively). In addition, the whiteness of TLP 2.5 was significantly (p<0.05) higher than TLP 11.5, with values of 55.09 and 44.83, respectively. These results showed that TLP 2.5 obtained following the acidic solubilization had a much lighter coloration than TLP 11.5 obtained following the alkaline solubilization. Similar results were also reported by Marmon & Undeland [2010] who found that the recovered proteins from gutted herring using the acidic pH shift process were lighter and whiter than those using the alkaline pH shift process which was probably due to the better removal of pigments, melanin, hemoglobin, and myoglobin. Additionally, Kristinsson & Rasco [2000] reported that the connective tissue present in the fish protein isolates may cause an increase in brightness.
The a* values of both TLP 2.5 and TLP 11.5 were decreased after the pH shift process, which contained 12.08 and 11.00, respectively (Table 1). However, TLP 2.5 had remarkedly (p<0.05) higher b* values than TLP 11.5 and the control (30.98, 19.70, and 19.95, respectively). Of note, TLP 2.5 showed significantly (p<0.05) higher a* and b* values than TLP 11.5. Marmon & Undeland [2010] and Kang et al. [2018] reported that the a* and b* values of the recovered proteins from gutted herring and green crab obtained following the acidic pH shift process were higher than those of the alkaline pH shift process, which was probably due to the lipid retention in the recovered proteins. In addition, the presence of high levels of heme proteins, or the denaturation and oxidation of hemoglobin, might affect the a* and b* values of the recovered protein [Kristinsson & Rasco, 2000]. Overall, these results were consistent with the appearance of TLP 2.5 which showed as being lighter, whiter, redder, and yellower than TLP 11.5 and the TL powder (Table 1).
Amino acid profile
The amino acid profile of the TL powder and TLPs (TLP 2.5 and TLP 11.5) has been summarized in Table 2. The major amino acids found in the TL powder were cysteine (13.24 g/100 g protein), glutamic acid (12.56 g/100 g protein), aspartic acid (8.53 g/100 g protein), leucine (7.52 g/100 g protein), alanine (6.27 g/100 g protein), and lysine (6.22 g/100 g protein), whereas the dominant amino acids found in both TLP 2.5 and TLP 11.5 were glutamic acid (11.99–12.45 g/100 g protein), cysteine (11.99–12.39 g/100 g protein), aspartic acid (9.39–9.50 g/100 g protein), leucine (7.89–8.14 g/100 g protein), lysine (5.49–6.36 g/100 g protein), and alanine (5.59–5.71 g/100 g protein). Among the non-essential amino acids, the results revealed that TLP 2.5 and TLP 11.5 were rich in glutamic acid and aspartic acid, and alanine which provided umami and sweet flavor, respectively [Han et al., 2019]. Thus, both TLP 2.5 and TLP 11.5 could likely be considered as enhancers of flavor in food.
Table 2
Amino acid profile (g/100 g protein) of tuna liver powder (control), tuna liver protein powders (TLPs) obtained following the pH shift process and FAO reference pattern for adults.
Amino acid | Control | TLP 2.5 | TLP 11.5 | Reference pattern [FAO, 2013] |
---|---|---|---|---|
Aspartic acid2 | 8.53±0.13b | 9.50±0.06a | 9.39±0.02a | |
Glutamic acid2 | 12.56±0.14a | 11.99±0.11c | 12.45±0.00b | |
Serine2 | 4.00±0.03c | 4.15±0.04b | 4.35±0.00a | |
Histidine*2 | 2.34±0.04b | 2.56±0.03a | 2.14±0.02c | 1.5 |
Glycine1 | 4.54±0.09a | 4.16±0.04c | 4.29±0.01b | |
Threonine*2 | 4.35±0.04b | 4.43±0.03b | 4.62±0.00a | 2.3 |
Arginine2 | 4.73±0.09c | 5.56±0.07b | 5.64±0.01a | |
Alanine1 | 6.27±0.06a | 5.59±0.05c | 5.71±0.01b | |
Tyrosine | 3.12±0.02b | 3.40±0.03a | 3.35±0.01a | |
Cysteine | 13.24±0.61a | 11.99±0.17b | 12.39±0.07b | |
Valine*1 | 5.14±0.04a | 5.01±0.04b | 5.02±0.00b | 3.9 |
Methionine*1 | 2.53±0.02b | 2.68±0.03a | 2.69±0.01a | 2.2 |
Phenylalanine*1 | 4.32±0.02b | 5.00±0.04a | 4.94±0.01a | 3.8 |
Isoleucine*1 | 4.30±0.02b | 4.43±0.04a | 4.43±0.01a | 3.0 |
Leucine*1 | 7.52±0.03c | 7.89±0.07b | 8.14±0.01a | 5.9 |
Lysine*2 | 6.22±0.04a | 6.36±0.06a | 5.49±0.01b | 4.5 |
Proline1 | 4.85±0.07a | 4.76±0.03a | 4.32±0.03b | |
Tryptophan*1 | 1.43±0.01a | 0.53±0.01c | 0.65±0.01b | 0.6 |
EAAs | 38.26±0.25b | 38.89±0.05a | 38.11±0.03b | |
HAAs | 40.90±0.15a | 40.05±0.09b | 40.18±0.04b | |
HPAAs | 42.73±0.27c | 44.56±0.05a | 44.08±0.08b |
2 Hydrophilic amino acid. TLP 2.5, TLP from solubilization at pH 2.5; TLP 11.5, TLP from solubilization at pH 11.5; EAAs, total essential amino acids; HAAs, total hydrophobic amino acids; HPAAs, total hydrophilic amino acids. Values are reported as mean ± standard deviation (n=3). Different letters within the same row indicate significant differences among treatments (p<0.05).
Of note, the essential amino acid (EAA) content in both TLP 2.5 and TLP 11.5 was higher and similar to the control (38.89, 38.11, and 38.26 g/100 g protein, respectively) (Table 2). The results agreed well with those of the previous study by Marmon & Undeland [2010] who reported that the content of EAA in the recovered proteins from gutted herring increased significantly during the pH shift processing. Comparing between the TLP groups, the EAA content of lysine and histidine in TLP 2.5 were markedly (p<0.05) higher, whereas the content of leucine, threonine, and tryptophan were significantly (p<0.05) lower than in TLP 11.5. The prominent EAAs of the TLP groups were leucine, lysine, and valine. Similar findings were also reported by Shen et al. [2022] who found that the most abundant EAAs in the recovered proteins from the liver of yellowfin tuna obtained following the acidic and alkaline pH shift processes were leucine, lysine, and valine. These EAAs are important as they promote brain functions and are associated with muscle metabolism and boost energy [Sarojnalini & Hei, 2019]. Overall, the content of all EAAs in both TLP 2.5 and TLP 11.5 meet the amino acid requirements for adults according to the suggestions of the Food and Agriculture Organization [FAO, 2013].
It is well-known that the nutritional and biological activities of protein are enhanced by the type, position in protein structure, and content of hydrophobic amino acid (HAA) and hydrophilic amino acid (HPAA) [Cha et al., 2020]. In the present study, according to the HAA content of all treatments ranging from 40.05 to 40.90 g/100 g protein, the predominant HAAs were leucine, alanine, and valine. On the other hand, the HPAA content obtained following all treatments ranged from 42.73 to 44.56 g/100 g protein where the major HPAAs were glutamic acid, aspartic acid, and lysine. A similar result was also observed by Cha et al. [2020] who found that the most remarkable HAAs in the recovered proteins from the roe of skipjack tuna obtained following the pH shift process were leucine, alanine, and valine, while the major HPAAs were glutamic acid and aspartic acid. Our results point out that the recovered proteins from skipjack TL obtained following the acid and alkaline pH shift processes could be considered a promising source of nutrients, particularly regarding amino acids.
Functional properties
Solubility of protein isolates
Solubility is an important functional property of proteins and protein-based formulations as it influences the usefulness of an ingredient in food and governs its physical characteristics [Freitas et al., 2011]. The solubility of TLP 2.5 and TLP 11.5 in the pH range of 2 to 12 is depicted in Figure 4. There were significant (p<0.05) differences in the protein solubility between TLP 2.5 and TLP 11.5 at pHs 3–4 and 6–8 by which TLP 2.5 had a higher protein solubility (5.58–13.36%) than TLP 11.5 (0.13–5.65%). This could be explained by the prominent amount of polar and hydrophilic amino acid residues, such as lysine and histidine, in TLP 2.5 compared to TLP 11.5 (p<0.05) (Table 2) which could have hydrophilic interaction with water and consequently promote protein solubility [Cha et al., 2020]. The highest solubility of TLPs was observed at pH 11 to 12 (38.41–42.45%). These results indicated that the extremely high alkaline conditions affected an increase in protein solubility which can be explained by the increasing net negative charges and hydrophilic amino acids leading to more binding sites for water [Kristinsson & Rasco, 2000]. However, both TLP 2.5 and TLP 11.5 showed minimum solubilities at pH 3–8 (0.13–13.36%) which could be explained by the proximity between the pH of the solution and the isoelectric point of the protein that could enhance protein precipitation. This result was consistent with the findings of Lone et al. [2015] and Cha et al. [2020] for rainbow trout and skipjack tuna roe protein which showed the lowest solubilities at pH 4–8 due to the proximity between the pH of the solution and the isoelectric point of the proteins. Generally, when the pH nearly reaches the isoelectric point, the protein net charge is minimized, resulting in protein aggregation [Lone et al., 2015].
Figure 4
Relative protein solubility (%) at pH 2.0 to 12.0 of tuna liver protein powders (TLP) obtained following the pH shift process. TLP 2.5, TLP from solubilization at pH 2.5; TLP 11.5, TLP from solubilization at pH 11.5; SP, soy protein concentrate; EW, egg white powder. SP and EW were used as positive controls. Values are reported as mean ± standard deviation (n=3).
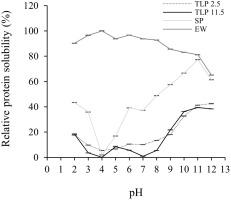
In this study, soy protein concentrate (SP) and egg white (EW) were used as positive controls, representing protein from plant and animal origins, respectively. Surprisingly, both TLPs showed a more similar pattern of protein solubility to SP which was totally different from that of EW (Figure 3). Compared to EW, TLP 2.5 and TLP 11.5 showed considerably (p<0.05) lower solubility at all pH values. The relatively low solubility of the TLPs might be due to the low solubility of the myofibrillar proteins of fish at pHs between 4.0 and 9.0 [Pires et al., 2012]. Overall, both TLP 2.5 and TLP 11.5 exhibited a similar solubility to SP, suggesting the probability of TLPs application in food products.
Emulsifying properties
The emulsifying activity index (EAI) and the emulsifying stability index (ESI) of TLP 2.5 and TLP 11.5 compared to SP and EW are presented in Table 3. The results showed that TLP 2.5 had a significantly (p<0.05) higher EAI and ESI than TLP 11.5 (16.00 and 13.79 m2/g, respectively; and 38.98, and 34.76 min, respectively). These results agreed well with the report by Panpipat & Chaijan [2017] who found that the recovered proteins of bigeye snapper head byproducts obtained following the acidic pH shift process were higher in EAI than those of obtained following the alkaline pH shift process. This was probably due to the partial unfolding of muscle protein induced by the acidic condition which was responsible for the incorporation of protein into the oil droplet membrane and the positive effect on emulsification [Panpipat & Chaijan, 2017]. However, the EAI of SP and EW was higher than that of TLP 2.5 and TLP 11.5. This might be due to the higher hydrophobicity of SP and EW which promotes the interaction of protein with the oil surface, resulting in excellent emulsifying capacity [Pires et al., 2012].
Table 3
Functional properties of tuna liver protein powders (TLPs) obtained following the pH shift process.
[i] TLP 2.5, TLP from solubilization at pH 2.5; TLP 11.5, TLP from solubilization at pH 11.5; SP, soy protein concentrate; EW, egg white powder; EAI, emulsifying activity index; ESI, emulsion stability index; FC, foaming capacity; FS, foaming stability; WHC, water holding capacity; OHC, oil holding capacity. SP and EW were used as positive controls. Values are reported as mean ± standard deviation (n=3). Different letters within the same row indicate significant differences among treatments (p<0.05).
The higher (p<0.05) ESI of TLP 2.5 than TLP 11.5 may be attributed to the capability of the emulsion droplets to be dispersed without coalescence, flocculation, and cream. This result agreed well with the finding of Shen et al. [2022] for yellowfin tuna liver protein which showed a higher ESI obtained following the acidic rather than the alkaline pH shift process. The ESI of both TLP 2.5 and TLP 11.5 was considerably (p<0.05) higher than EW. This could be explained by the revelation of hydrophobic groups under the acidic and alkaline pH shift processes which stabilized the protein network formed and consequently led to the stability of the emulsion [Panpipat & Chaijan, 2017; Cha et al., 2020]. However, the ESI of the TLPs was significantly (p<0.05) lower than that of SP. This might be correlated with the lower EAI of the TLPs.
Foaming properties
The foaming capacity (FC) and the foaming stability (FS) of the TLPs are shown in Table 3. The FC of TLP 11.5 was remarkably (p<0.05) greater than those of TLP 2.5 with values of 126.67% and 113.33%, respectively. In addition, the FS of TLP 11.5 was significantly (p<0.05) higher than that of TLP 2.5, which were 84.44% and 53.12%, respectively. The results for the FC from TLP 11.5 were similar to those reported by Shen et al. [2022] for the recovered proteins from yellowfin tuna liver through which the alkaline pH shift process showed a higher FC than the acidic pH shift process. This might be due to the exposure to a larger number of hydrophobic groups by the alkaline pH shift process which led to the better FC of the protein [Cha et al., 2020; Shen et al., 2022]. Additionally, the FS of TLP 11.5 was similar to that reported by Chanted et al. [2022] for the pig brain proteins obtained following the alkaline pH shift process which showed an excellent FS compared to the acidic pH shift process.
Both TLP 2.5 and TLP 11.5 had a significantly (p<0.05) lower FC than SP and EW (Table 3). This might be related to the protein solubility of the TLPs showing as being lower than SP and EW (Figure 4), which affected its ability to unfold at the air-water interface [Cha et al., 2020]. This result was in accordance with the finding of Pires et al. [2012] who reported that the hake protein powder showed a lower FC than EW could be related to the lower protein solubility of the fish proteins. Interestingly, the FS of TLP 11.5 was greater than 80% which was comparable to that of SP and EW, indicating its potential as an excellent foam stabilizer. According to these results, the TLP prepared by the alkaline pH shift process possessed a high potency for foam stability which may be applicable in foam-based foods.
Water holding capacity
The water holding capacity (WHC) of TLPs is shown in Table 3. The WHC of TLP 11.5 was significantly (p<0.05) higher than that of TLP 2.5, accounting for 18.61 and 8.06 mL/g, respectively. These results agree well with Freitas et al. [2011] who found that the recovered protein of Argentine anchovy (Engraulis anchoita) obtained by the alkaline pH shift process had a higher WHC than the recovered proteins obtained following the acidic pH shift process. The lower WHC of TLP 2.5 might be related to the higher protein solubility (Figure 4) caused by the higher content of the polar group in the proteins which decreases the amount of adsorbed water [Han et al., 2019]. The WHC of both TLPs was significantly (p<0.05) lower than that of SP but significantly (p<0.05) higher than that of EW. This might be due to the conformational changes of the TLPs caused by the acidic and alkaline pH shift processes which allowed accessibility between the hydrophilic amino acids and water, leading to the increasing WHC [Cha et al., 2020].
Oil holding capacity
The oil holding capacity (OHC) of the TLPs is shown in Table 3. The OHC is an important characteristic required in the meat and emulsions industry as it affects the taste and functional properties of food [Freitas et al., 2011]. The results showed that TLP 11.5 had a higher (p<0.05) OHC than TLP 2.5 with values of 8.67 and 5.73 mL/g, respectively. The high OHC of TLP 11.5 may be due to the larger amount of hydrophobic amino acids (i.e., cysteine, alanine, glycine, and tryptophan) existing on the protein surface [Han et al., 2019]. Additionally, both TLPs exhibited a higher (p<0.05) oil holding capacity than EW which may be related to the differences in protein content and hydrophobic amino acids such as valine, tryptophan, and phenylalanine, which can easily bind to oil [Han et al., 2019].
Overall, the functional property analyses of the TLPs obtained through the acidic and alkaline pH shift process pointed out that these proteins are equivalent in many aspects to commercial proteins such as SP and EW, particularly in terms of solubility, emulsion properties, and water/oil holding capacity. Thus, TLPs could potentially be applied in various food systems and could potentially be used as alternative emulsifiers and in water/oil adsorption as part of emulsion-based food products.
Antioxidant properties
DPPH radical scavenging activity
DPPH• is a stable free radical which can accept an electron or hydrogen radical and form a stable molecule [Yen & Hsieh, 1995]. It is widely used for the evaluation of the radical scavenging activity of primary antioxidants. In this study, the DPPH radical scavenging activity of both TLP 2.5 and TLP 11.5 are shown in Figure 5A. TLP 11.5 showed a significantly (p<0.05) higher activity than TLP 2.5, accounting for 0.73 and 0.55 µmol Trolox equivalent/mg sample, respectively. According to Zhang et al. 2018], the higher DPPH radical scavenging activity could be explained by the presence of hydrophobic amino acids or aromatic amino acids (alanine, leucine, valine, and isoleucine) in the recovered proteins obtained following the alkaline pH shift process. The pH shift process is based on acidic and alkaline solubilization and isoelectric precipitation of proteins [Kristinsson et al., 2005]. Since alanine, leucine, and isoleucine are more soluble in alkaline pH [Tseng et al., 2009], the higher scavenging activity of TLP 11.5 coincides with the significantly (p<0.05) higher leucine and alanine contents (Table 2) which were responsible for the DPPH radical scavenging activity [Zhang et al., 2018]. Our TLP 11.5 showed higher DPPH radical scavenging activity under the same sample concentration compared to the cuttlefish (Sepia pharaonis) protein isolates obtained from alkaline pH shift process [Hamzeh et al., 2018].
Figure 5
Antioxidant and angiotensin I-converting enzyme (ACE) inhibitory activities of tuna liver protein powders (TLP) obtained following the pH shift process. DPPH radical scavenging activity (A), ABTS radical cation scavenging activity (B), ferrous-ion chelating activity (C), and ACE inhibitory activity (D). Values are reported as mean ± standard deviation (n=3). Different letters indicate significant difference among treatments (p<0.05).
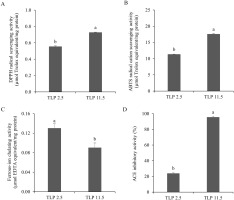
ABTS radical cation scavenging activity
Value of ABTS radical cation scavenging assay is well accepted as an antioxidant index due to the applicability of both lipophilic and hydrophilic compounds [Cha et al., 2020]. The ABTS•+ scavenging activity of the TLPs are shown in Figure 5B. The ABTS•+ scavenging activity of TLP 11.5 was 17.56 µmol Trolox equivalent/mg protein, which was greater (p<0.05) than that of TLP 2.5 (11.32 µmol Trolox equivalent/mg protein). This could be attributed to the higher amounts of some hydrophilic amino acids, specifically glutamic acid, arginine, and threonine in TLP 11.5 compared to those in TLP 2.5 (Table 2). These amino acids typically exhibited high ABTS•+ scavenging activity [Cha et al., 2020]. Additionally, the alkaline condition can induce the generation of negatively charged peptides [Binsan et al., 2008] which could in turn promote the ABTS•+ scavenging activity of TLP 11.5. A similar phenomenon was observed by Cha et al. [2020] who reported that the roe tuna recovered protein from the alkaline pH shift process exhibited high ABTS•+ scavenging activity. Compared to the rainbow trout by-product protein isolates obtained following alkaline pH shift process by Nikoo et al. [2019], TLP 11.5 showed greater ABTS•+ radical scavenging activity under the same sample concentration.
Ferrous-ion chelating activity
Ferrous-ion chelating activity is one of the most important indicators for determining the antioxidant properties of compounds. This activity is based on the bonding between the ferrous iron and functional carboxyl, amino, and hydroxy groups in antioxidants [Torres-Fuentes et al., 2012]. The ferrous-ion chelating activity of the TLPs is shown in Figure 5C. Unlike the other antioxidant properties, namely the DPPH• and ABTS•+ scavenging activities, TLP 2.5 exhibited a greater ferrous-ion chelating activity than TLP 11.5 (0.13 and 0.08 µmol EDTA equivalent/mg protein, respectively). This could be explained by the higher (p<0.05) content of polar amino acids (lysine and histidine) and other amino acids (proline) in TLP 2.5 than in TLP 11.5 (Table 2) which was implicated in its high iron-binding capacity [Torres-Fuentes et al., 2012]. Sun et al. 2020] also reported a positive correlation between ferrous-ion chelating activity and histidine content which was higher in TLP 2.5 in this study. In addition, the NaCl content could affect the ferrous-ion chelating activity of protein by disrupting the chelating activity of specific peptides and amino acid side chain groups in protein [Zhu et al., 2014]. In the present study, the content of NaCl in TLP 11.5 was higher than in TLP 2.5. These resulted in the lower ferrous-ion chelating activity in TLP 11.5 than in TLP 2.5. However, TLP 11.5 still showed greater ferrousion chelating activity under the same sample concentration compared to the shrimp waste protein isolates obtained following pH shift process [Khumallambam et al., 2011].
ACE inhibitory activity
The inhibition of ACE, a key enzyme involved in the regulation of blood pressure, is an important pharmacological target to treat hypertension. Currently, ACE inhibitory peptides derived from food proteins have been well accepted as safe and excellent ACE inhibitors [Cha et al., 2020]. The ACE inhibitory activity of both TLP 2.5 and TLP 11.5 is depicted in Figure 5D. The results showed a significantly (p<0.05) higher ACE inhibitory activity in TLP 11.5 (95.66%) than TLP 2.5 (24.03%) (0.13 and 2.28 mmol Captopril/mg sample, respectively). This is probably due to a higher content of some hydrophobic amino acids, such as leucine, and alanine, and some hydrophilic amino acids, such as arginine in TLP 11.5 than TLP 2.5 (Table 2). Similar results have been reported by Nakajima et al. [2009] according to whom the higher percentages of alanine and leucine, and to some extent methionine, led to the strong ACE inhibitory activity of fish muscle hydrolysate. The result obtained in the present study was in accordance with the findings of Cha et al. [2020] who reported that the ACE inhibitory activity of the recovered proteins from roe yellowfin tuna prepared by the alkaline pH shift process exhibited high ACE inhibitory activity which was probably due to the present of alanine and arginine. The alkaline pH shift process also affected the cleavage of the inter- and intra-molecular hydrogen bonds within and between the molecules which led to the faster release of ACE inhibitory peptides [Zhang et al., 2017]. Furthermore, Kim et al. [2016] reported that the existence of NaCl could enhance the ACE inhibitory activity of peptides leading to the higher ACE inhibitory activity of TLP 11.5 than that of TLP 2.5. In comparison to the roe tuna protein isolates obtained following alkaline pH shift process by Cha et al. [2020], TLP 11.5 showed greater ACE inhibitory activity under the same sample concentration.
The results obtained in the present study indicate that TLP from the alkaline pH shift process (TLP 11.5) possessed better antioxidant activities, especially DPPH• and ABTS•+ scavenging activities, and ACE inhibitory activity than those obtained following the acidic pH shift process (TLP 2.5). Hence, the alkaline pH shift process is an excellent method for the development of TLP as a natural antioxidant and ACE inhibitor.
CONCLUSIONS
This study demonstrated the feasibility of producing recovered protein powder derived from valueless skipjack tuna liver through an acidic or alkaline pH shift process (TLP 2.5 or TLP 11.5). The protein recovery yield was higher in alkaline conditions (pH 11.5) than in acidic conditions (pH 2.5). Lipids were efficiently removed by the acidic and alkaline pH shift process. Both TLP 2.5 and TLP 11.5 were rich in essential amino acids, especially leucine, lysine, and valine. Compared with TLP 2.5, the TLP 11.5 from the alkaline pH shift process possessed better foaming properties and water/oil holding capacity. In addition, the TLP 11.5 had stronger bioactive properties, in terms of DPPH• and ABTS•+ scavenging activities, and ACE inhibitory activity. Therefore, the TLP obtained by the alkaline pH shift process could potentially be applicable as an alternative functional protein ingredient. Further studies on the characterization of bioactive components responsible for antioxidant and ACE inhibitory activities could be valuable for TLP as nutraceutical and functional food ingredients for protein food and pharmaceutical applications.