INTRODUCTION
Citrus fruits, renowned for their nutritional richness and distinctive flavors, have become a staple food in global consumption patterns. Among these, oranges stand out as a particularly valuable source of essential bioactive compounds, including polyphenols, carotenoids, ascorbic acid, and limonoids, contributing not only to their distinct taste and aroma but also to a myriad of health benefits [Liu et al., 2022; Lv et al., 2015]. Despite their appeal, fresh oranges face perishability issues due to a high water content of 87% [Omar & Matjafri, 2013]. In response to this challenge, various processing techniques, such as dehydration, have been explored to extend shelf life, and at the same time reduce packaging, storage, and transportation costs [Sun et al., 2019]. Osmotic dehydration (OD) emerges as a pivotal method in this context, distinguished for its cost-effectiveness and unique mass transfer dynamics [Abrahão & Corrêa, 2023]. This technique involves immersing food in hypertonic solutions, creating counter-current flows that facilitate the removal of water from the tissue to the surrounding solution and the diffusion of osmotic solutes into the tissue, effectively preserving the food product [Ahmad, 2023]. As a non-thermal processing technique, OD has gained favor in recent years due to increasing consumer demand for fresh, nutritious foods and the need for energy-efficient and environmentally friendly technologies [Abrahão & Corrêa, 2023]. OD offers several advantages, including the preservation of flavor and color, suppression of enzyme browning, and reduced energy consumption [Abrahão & Corrêa, 2023]. The effectiveness of OD is influenced by various parameters, such as the type of osmotic agent, concentration of hypertonic solution, agitation, temperature, immersion time, sample-to-solution ratio, and the characteristics of the material undergoing the process [Ahmad, 2023]. The concentration of the osmotic agent solution plays a crucial role in impacting mass transfer kinetics; with higher concentrations resulting in faster osmosis rates. This concentration-dependent effect influences the rate of water loss and solid gain during extended osmotic treatment [Salehi et al., 2023].
The most commonly used osmotic agents for OD of fruits and vegetables are sucrose, invert sugar, sodium chloride, corn syrup, and combinations thereof [Yadav & Singh, 2014]. Recently, natural sweeteners and by-products of the sugar industry have also been increasingly studied in this aspect, including honey, jaggery, sugar beet molasses and cane molasses [Kaur et al., 2022]. Other unconventional osmotic agents successfully used in fruit dehydration were concentrated fruit juices and fruit syrups [González-Pérez et al., 2021; Kowalska et al, 2023]. Because these osmotic agents contain many nutritional and bioactive compounds in addition to sugars, they positively influence the nutritional, health-promoting and sensory quality of dried fruits.
The edible fruit of prickly pear cactus is popular in Mexico and in the Mediterranean region. It contains sugar (15 g/100 g) and other nutrients and bioactive compounds including protein, minerals, amino acids, vitamins, flavonoids, and betalains [Cota-Sánchez, 2016; Jimenez-Aguilar et al., 2014]. In our previous study, the molasses (sugar syrup) from prickly pear by-products with a superior sugar level (39.93°Brix) and antioxidant capacity was obtained [Yazidi et al., 2024]. Its formulation was optimized by using statistical modeling, and the optimal cooking conditions were established at 78.35°C for 79.70 min, with the following proportion of ingredients: 0.265 g of pulp, 0.710 g of peel, and 0.025 g of seed. In this study, we proposed the pioneering use of prickly pear molasses as an osmotic agent.
Against this backdrop, the aim of this study was to characterize the osmotic dehydration of orange fruit slices in prickly pear molasses and sucrose solutions. The research not only explored the changes in total mass loss, water loss and solid gain during the process, but also entailed an in-depth analysis of the chemical and physical properties of the obtained material, comparing it with fresh orange slices.
MATERIAL AND METHODS
Preparation of raw material and osmotic solution
Fresh Navelina oranges (Citrus sinensis var. Navelina) were taken from Polish company which imported them from Spain. Oranges were washed and cut into 3-mm slices for OD. Two osmotic agents, sucrose and prickly pear molasses, were employed. To obtain molasses, prickly pear (Opuntia ficus-indica) fruits were harvested in August 2022 from naturalized plantations in Sbikha, Kairouan, Tunisia. Fruits were selected at the 50% color-break stage to ensure uniform ripeness and optimal sugar content. Prickly pear fruit underwent a cleaning process to remove spines, cut two sides, and the remaining fruit was divided into four parts. Equal parts of fruit and water were heated for about 1 h, filtered through a 2-mm sieve, and the filtrate was concentrated to a soluble solid content of 70°Brix.
Osmotic dehydration
The OD treatment was conducted according to procedure described by Nowacka et al. [2014]. The orange slices were submerged in a 70°Brix osmotic agent solution (sucrose or molasses) with a mass ratio of 1:4. The process was carried out at 30°C for 3 h in a glass vessel placed in a shaking water bath. After 0.5, 1, 2, and 3 h, the samples were removed from the solution using a metal sieve and then dried for 10 s on both sides on filter paper. The mass transfer dynamic during OD was assessed by determining total mass loss (TML), water loss (WL), and solid gain (SG). TML of the sample underwent a reduction due to water loss and at same time weight increased due to a solute gain. Thus, TML represents the difference between water loss and solid gain. WL (kg/kg) was defined as the water loss of the sample during OD on fresh sample weight basis and was calculated using Equation (1):
SG (kg/kg) was the total gain of sugar and other solids by the sample on fresh sample weight basis, and was calculated using Equation (2):
where: M0 is the water content of a fresh sample (kg), Mi is the water content of an osmotically dehydrated sample (kg), S0 is the solid content of a fresh sample (kg), Si is the solid content of an osmotically dehydrated sample (kg), and W0 is the total weight of a fresh sample (kg).The moisture content was determined using AOAC International method by drying the fresh and osmotically dehydrated orange slices in a Binder FP115 oven (Binder, Tuttlingen, Germany) at 105°C until constant weight [AOAC, 2002]. The entire OD process was conducted in duplicate.
Determination of physical properties
Dry matter content determination involved both fresh and osmotically dehydrated orange slices after 0.5, 1, 2, and 3 h, employing the gravimetric method with drying at 70°C [da Silva et al., 2014]. Water activity measurements were conducted at room temperature in triplicate for each sample using the AquaLab Series 3TE water activity meter (Decagon Devices, Inc., Pullman, WA, USA). Color on the sample surface was quantified using a colorimeter (Model CR-300, Minolta, Osaka, Japan), examining parameters such as L* (lightness), a* (redness/greenness), b* (yellowness/blueness), hue angle (H) in ten replications. The total color difference (ΔE) was calculated using Equation (3):
where: ΔL*, Δa* and Δb* are the differences between the individual color parameters of dehydrated orange slices and the corresponding color parameters of fresh orange slices.Texture properties were assessed through a penetration test using a TA-HDi500 texture analyzer (Stable Micro Systems, Surrey, UK), performing at least 10 replicates for each sample. The maximum force (hardness, N) needed to penetrate the orange slices, and compression work (mJ) were recorded.
Total soluble solids, expressed in °Brix, were measured directly using a refractometer (PAL-3, Atago Co., Ltd., Tokyo, Japan).
Microstructure imaging
The microstructure of fresh and osmotically dehydrated for 3 h orange slices was imaged as described by Kowalska et al. [2023]. Samples were affixed to a metallic table and coated with a 5-mm layer of gold using the Leica EM ACE200 system (Leica Microsystems GmbH, Vienna, Austria). Cross-sectional examinations were conducted employing a Phenom XL scanning electron microscope (Thermo Fisher Scientific, Waltham, MA, USA), operating at an accelerating voltage of 10 kV and a chamber pressure of 60 Pa. A minimum of four images were captured for each cross-section, and at least six images were obtained for the surface of each sample at a magnification of 500×.
Total phenolic content and antioxidant capacity determinations
The analyses encompassed extraction, total phenolic content (TPC) determination, and assessment of antioxidant capacity. The extraction procedure, adapted from Nowacka et al. [2019], involved crushing each orange slice sample, followed by combining 300 mg of the sample with 10 mL of an 80% (v/v) ethanol solution and 0.1 M HCl (85:15, v/v). After 12 h of extraction with continuous shaking and subsequent centrifugation, the supernatant was collected for analysis. TPC in extracts and hypertonic solutions was evaluated using the Folin–Ciocalteu reagent and a chlorogenic acid (CA) standard curve. Results were expressed as mg CA/100 g dry matter (DM) of the sample [Chun & Kim, 2004].
Antioxidant capacity was measured as ABTS•+ scavenging activity and reducing power. The assay with 2,2’-azino-bis-(3-ethylbenzothiazoline-6-sulfonic acid) (ABTS) was performed using the method developed by Re et al. [1999]. The absorbance of the reaction mixture with samples and generated ABTS•+ was measured at a wavelength of 734 nm. The results were expressed as mg Trolox /g DM based on a calibration curve determined from this standard (100–1,000 μM). The reducing power of the samples was determined according to the procedure with potassium ferricyanide and FeCl3 described by Oyaizu [1986]. The absorbance was measured at a wavelength of 700 nm. The results were expressed as mg Trolox /g DM. All analyses were conducted in triplicate.
Ascorbic acid content determination
Ascorbic acid content of fresh and osmotically dehydrated for 3 h orange slices was determined using the H-Class ultra-performance liquid chromatography (UPLC) system with a photodiode array detector (Waters, Milford, MA, USA) [Kowalska et al., 2023]. Crushed material (0.05 g) was extracted with 10 mL of a solution of 3% meta-phosphoric acid, 8% acetic acid, and 1 mM EDTA (v/v/v) for 10 min, followed by centrifugation (6,000×g, 5°C, 5 min). The filtered supernatant was analyzed after a 2-fold dilution with a 0.1% formic acid solution in Milli-Q water. Separation was conducted on a Waters HSS T3 column (100×2.1 mm, 1.8 μm). The flow rate of the mobile phase (0.1% formic acid) was 0.25 mL/min, the injection volume was 5 μL, and the temperatures of the column thermostat and samples were 25 and 4°C, respectively. The absorbance recorded at 245 nm was used to quantify ascorbic acid in orange slices. Results were expressed as mg/100 g DM of the sample based on the calibration curve plotted for the ascorbic acid standard.
Sugar content determination
The high-performance liquid chromatography (HPLC) Spectra-SYSTEM with a P100 pump, a refractive index detector and a Rheodyne injection valve with a 20 μL-sample loop (Waters, Milford, MA, USA) was employed for sugar content determination in fresh and dehydrated orange slices. Separation occurred on a Sugar-Pak I column (300×6.5 mm; Waters) at 90°C, with a mobile phase (Milli-Q water) flow rate of 0.6 mL/min [Yang et al., 2021]. The homogenized material (approximately 0.3 g in triplicate) was suspended with 10 mL of water at 80°C, extracted at 25°C for 4 h, and after filtration, analyzed by HPLC. Standard solutions containing the reference compounds (fructose, glucose and sucrose) were prepared in milli-Q water. Retention time of standards was used to identify individual sugars in the samples. Quantification was based on the calibration curves plotted for standards. Results were expressed as mg/100 g DM of orange slices.
Total carotenoid content determination
The spectrophotometric method was employed to determine the total carotenoid content in the orange samples. The extraction process involved two stages: initially, with 100% acetone, followed by the addition of petroleum ether (100%), a more specific solvent for carotenoid extraction, into the mixture, while the acetone fraction was discarded [Rodriguez-Amaya, 2001]. The absorbance of the collected petroleum ether fraction was measured at 450 nm. The total carotenoid content was quantified and expressed as mg of β-carotene equivalent per kg of sample DM.
Statistical analysis
Results reported in this work are presented as mean of three independent values accompanied by standard deviation. Experimental data were analyzed using JMP 14 software (SAS Institute Inc., Cary, NC, USA). Analysis of variance (ANOVA) was performed and Tukey-Kramer comparison test was used to estimate significant differences through osmotic dehydration time (p<0.05). Student’s t-test was used to show significant difference (p<0.05) between osmotically dehydrated orange slices and fresh orange slices.
RESULTS AND DISCUSSION
Osmotic dehydration of orange fruit slices in sucrose and molasses solutions
In the process of OD, water migrates from the food matrix into the hypertonic solution, while solutes from the solution permeate into the food matrix. This phenomenon is driven by the variance in osmotic pressure between the food tissue and the osmotic solution [Abrahão & Corrêa, 2023]. The changes in total mass loss, water loss, solid gain, and the ratio of water loss to solid gain for slices of orange fruits during OD in sucrose and molasses solutions demonstrated distinctive patterns (Table 1). In sucrose, total mass loss steadily increased from 0.07 to 0.16 kg/kg between 0.5 and 3 h of processing. Immersion in molasses escalated total mass loss from 0.07 to 0.18 kg/kg during the same processing period, significantly (p<0.05) exceeding the values recorded for the samples dehydrated in the sucrose solution at each time point after 0.5 h of the dehydration, suggesting a faster process possibly due to higher osmotic potential. The orange slice water loss increased gradually from 0.13 kg H2O/kg at 0.5 h to 0.26 kg H2O/kg at 3 h in the sucrose solution, suggesting an effective dehydration process. Similarly, immersion in the molasses solution demonstrated an escalating water loss from 0.08 kg H2O/kg at 0.5 h to 0.25 kg H2O/kg at 3 h. Solid gain of orange slices dehydrated in the sucrose solution increased progressively from 0.06 kg/kg to 0.11 kg/kg, while that recorded for samples dehydrated in molasses, although following a similar trend, consistently displayed lower values, implying that the sucrose solution induced a more significant solid gain. The water loss to solid gain ratio during the OD of orange fruits in sucrose and molasses solutions provided insights into the efficiency of the dehydration process. In sucrose, the ratio ranged from 2.12 to 2.36 without significant (p≥0.05) differences between dehydration time points, indicating that for each unit of solid gained, approximately 2.12 to 2.36 units of water were lost. On the other hand, in molasses, the ratio decreased from 4.01 at 0.5 h to 3.12 at 3 h, suggesting a higher water loss for each unit of solid gained compared to immersion in the sucrose solution.
Table 1
Mass loss, water loss, solid gain and physicochemical characteristics of orange fruits during their osmotic dehydration in sucrose and prickly pear molasses solutions.
[i] Results are shown as mean ± standard deviation (n=3). Different letters a–d in rows indicate significant differences between the samples at different dehydration times (p<0.05). Different A and B letters indicate significant differences between treatments separately for each dehydration time and parameter (p<0.05).
Molasses notable osmotic potential has made it a preferred choice for enhancing the dehydration process in food applications [Filipović et al., 2022; Nićetin et al., 2022; Šuput et al., 2020]. El Hosry et al. [2023] reported that fruit molasses has the complex composition. This complex composition of molasses solutions may be the reason for a higher rate of total mass loss of the dehydrated material compared to dehydration in a sugar solution, which was observed in our study during OD of orange fruit. Molasses was produced from prickly pear fruit that contains not only sucrose but also other solutes, such as organic acids, minerals, and non-sugar organic compounds [Belviranlı et al., 2019; Tsegay, 2020]. This diverse composition contributes to a higher osmotic potential in molasses compared to a simple sugar solution.
Impact of osmotic dehydration on the water activity of orange slices
Fresh orange fruit exhibited a water activity of 0.947 (Figure 1), indicating high water availability for biological and chemical reactions. No statistically significant (p≥0.05) difference existed between a water activity of orange slices dehydrated for 3 h in the sucrose solution (0.895) and prickly pear molasses (0.909), indicating comparably reduced water availability in these solutions vs. fresh fruit. Kowalska et al. [2023] noted that fruit juice concentrates (from chokeberry, strawberry and cherry) as osmotic agents significantly reduced water activity, with the most substantial decrease observed in strawberries dehydrated in cherry concentrate (0.873). Sucrose and molasses solutions, with lower water activity, contributed to food preservation by inhibiting microbial growth and enzymatic reactions [Gomez et al., 2021]. Understanding these values is crucial for assessing the impact of different solutions on food system stability and shelf life [Tapia et al., 2020].
Impact of hypertonic solution type on total soluble solid and dry matter contents of orange slices during osmotic dehydration
The trends in changes in the total soluble solid content of orange slices during fruit OD in sucrose and molasses solutions are shown in Table 1. During OD in the sucrose solution, the content of total soluble solids increased continuously from 17.53°Brix at 0.5 h to 25.83°Brix at 3 h, indicating ongoing solute accumulation in orange slices. The total soluble solids of fruits dehydrated in molasses showed a similar trend, with values increasing from 18.18°Brix at 0.5 h to 28.06°Brix at 3 h, suggesting that prickly pear molasses was a more concentrated solute environment than sucrose. Compared to the total soluble solids of fresh orange fruit (10.00°Brix), OD resulted in a notable increase in solute content. As the total soluble solid content of orange slices increased over dehydration time in both sucrose and molasses solutions, a corresponding increase in dry matter content was found, showcasing the absorption of substances dissolved in hypertonic solutions by the orange fruit. Rubio-Arraez et al. [2015] also studied the effect of isomaltulose, oligofructose and aqueous extract of stevia solutions on total soluble solid contents of orange slices during osmotic dehydration and they found that the content of soluble solids in orange slices augmented in conjunction with the elongation of the dehydration time.
Impact of osmotic dehydration on orange fruit color
Results of color analysis of fresh orange slices and these osmotically dehydrated in the sucrose solution and prickly pear molasses for 3 h, depicted by L*, a*, b*, ΔE and H° values, are shown in Table 2. Fresh orange slices were characterized by L*, a*, and b* color parameters at the levels of 44.69, −1.64, and 22.15, respectively. The L* increased gradually from 44.80 at 0.5 h to 47.50 at 3 h for orange slices dehydrated in the sucrose solution, while immersion in the molasses solution caused a decrease of L* from 39.50 at 0.5 h to 31.20 at 3 h. Similarly, the b* increased gradually from 22.40 at 0.5 h to 23.50 at 3 h for orange slices dehydrated in the sucrose solution, while decreased from 26.40 at 0.5 h to 23.02 at 3 h upon slices immersion in the molasses solution. For a*, there was an increase from −3.50 at 0.5 h to −2.50 at 3 h in the sucrose solution and from 3.10 at 0.5 h to 11.90 at 3 h in the molasses solution. According to Kowalska et al. [2023], the type of osmotic agent had a significant impact on the absolute color difference. Orange slices dehydrated in a molasses solution had a darker color compared with those dehydrated in a sucrose solution. This was related to the penetration of colored substances contained in the molasses solution into the fruit. Immersion in the sucrose solution resulted in minimally perceptible color changes of orange slices compared to fresh fruits, indicated by low ΔE values (3.28) and consistent hue values (Table 2), suggesting stable color tone. In contrast, orange slices dehydrated in molasses showed more pronounced color transformations with higher ΔE values (19.12) and a significant shift in hue from 94.23° (fresh fruits) to 62.75° (dehydrated fruits). These results suggested that molasses induced more substantial and perceptible color alterations in the orange fruit compared to sucrose, possibly due to the complex composition of molasses, including non-sugar components [Samborska et al., 2019]. These findings highlight the importance of considering color changes as part of the quality assessment for orange fruits osmotically dehydrated in different solutions.
Table 2
Color parameters of orange fruits during their osmotic dehydration in sucrose and prickly pear molasses solutions.
[i] Results are shown as mean ± standard deviation (n=3). Different letters a–d in rows indicate significant differences between the samples at different dehydration times (p<0.05). Different A and B letters indicate significant differences between treatments separately for each dehydration time and parameter (p<0.05). L*, lightness; a*, redness/greenness; b*, yellowness/ blueness; H°, hue angle; ΔE, total color difference compared to fresh orange slices.
Impact of osmotic dehydration on orange slice texture parameters and microstructure
Texture, a key quality parameter, was assessed in osmotically dehydrated orange fruits after 3 h in various solutions (Figure 2). Immersion in both sucrose and molasses solutions reduced hardness of orange slices to 0.52 N and 0.40 N, respectively, compared to the fresh state (0.63 N). Compression work of dehydrated orange slices was also lower (in sucrose solution – 0.38 mJ and in molasses solution – 0.36 mJ) compared to that of the fresh orange slices (0.58 mJ), reflecting a softer texture.These findings were consistent with the results reported by Kowalska et al. [2023] for strawberries osmo-dehydrated in fruit juice concentrates as hypertonic solutions. Additionally, Gamboa-Santos et al. [2021] revealed that OD of strawberries resulted in reduced elasticity and increased hardness due to the concentration and penetration of osmotic substances.
Figure 2
Texture parameters of fresh orange slices and these dehydrated in sucrose and prickly pear molasses solutions; maximum force (A) and compression work (B). Different letters above bars indicate statistical differences (p<0.05).
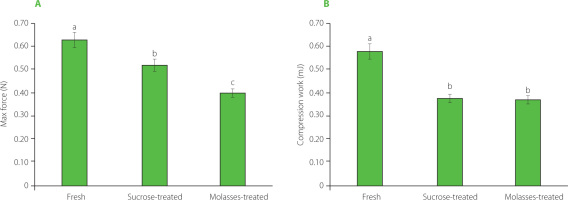
Microstructural analysis highlighted regular cellular arrangements in fresh slices, while dehydration in molasses induced significant tissue alterations, forming new crystals (Figure 3). This phenomenon was attributed to the substantial difference in osmotic pressure between the hypertonic solution and the rich composition of molasses, including non-sugar constituents. The distinctive microstructural changes emphasized the profound impact of solution choice on the physical characteristics of osmotically dehydrated orange fruits.
Effect of osmotic dehydration on total phenolic content and antioxidant capacity of orange slices
The total phenolic content and antioxidant capacity of fresh and dehydrated orange slices are shown in Table 3. Immersion in both sucrose and molasses solutions caused that the total phenolic content significantly (p<0.05) decreased compared to fresh slices, for which TPC was 2,536 mg CA/100 g. In fact, the sucrose-treated slices had 1,512 mg CA/100 g DM, while the molasses-treated ones had 2,197 mg CA/100 g DM. Devic et al. [2010] stated that the main mechanism responsible for decreasing phenolic compound content in fruits during OD is water diffusion, because water-soluble phenolics can be leached out together with the water flowing from the dehydrated plant material to the hypertonic solution. Another phenomenon that may occur during OD and cause the loss of some individual phenolics is their degradation by hydrolysis resulting in lower molecular weight molecules that can more easily pass through the cell membrane into the hypertonic solution [Almeida et al., 2015].
Table 3
Total phenolic content and antioxidant capacity of fresh orange slices, orange slices after 3-h osmotic dehydration in a sucrose solution and pearly pear molasses, and hypertonic solutions.
Antioxidant capacity, evaluated through ABTS and reducing power assays, showcased the molasses-treated slices with higher ABTS•+ scavenging activity (6.26 mg Trolox/g DM) than the sucrose-treated ones (2.68 mg Trolox/g DM). However, the sucrose-treated slices displayed greater reducing power (2.32 mg Trolox/g DM) than these immersed in molasses (1.74 mg Trolox/g DM). Similar results were obtained by Nićetin et al. [2022], who reported that the antioxidant capacity determined by ABTS and reducing power assays were increased after OD treatment of celery root in sugar beet molasses (1.10–1.13 mM Trolox/L and 1.54–1.58 mM Fe2/L, respectively) compared to the fresh samples (1.01 mM Trolox/L and 1.52 mM Fe2/L, respectively). They also found a strong positive correlation between the total phenolic content and antioxidant capacity in the ABTS assay (0.86, p≤0.01) and reducing power (0.79, p≤0.01). The composition of the osmotic solution has a direct effect on the retention of bioactive compounds in the dehydrated material during OD and, consequently, on its antioxidant capacity [Giovanelli et al., 2012]. Moreover, Almeida et al. [2015] who osmotically dehydrated banana slices in sucrose solutions, found that high concentrations of the osmotic solution resulted in a protective effect on phenolics, mainly tannins, and higher retention of antioxidant capacity. The authors concluded that the incorporation of solute into the banana tissue created a barrier to leaching of soluble solids, including phenolic compounds.
Impact of osmotic dehydration on content of ascorbic acid, total carotenoids, and sugars
The contents of ascorbic acid, total carotenoids and sugars in fresh orange slices and following 3 h of OD in various solutions are displayed in Table 4. Statistical analysis uncovered significant (p<0.05) differences in ascorbic acid content among dehydrated orange slices. Specifically, the molasses-treated slices exhibited significantly higher ascorbic acid content, at 50.14 mg/100 g DM, in contrast to the fresh slices (42.77 mg/100 g DM) and the sucrose-treated slices (27.20 mg/100 g DM). This indicates that molasses played a protective role in preserving ascorbic acid during dehydration, possibly due to its significant antioxidant capacity. Kowalska et al. [2023] used different fruit juice concentrates as osmotic agents and found the highest vitamin C content in fruits dehydrated in the strawberry juice concentrate (220.48 mg/100 g DM), and the lowest one in strawberries dehydrated in a sucrose solution (65.60 mg/100 g DM). However, the vitamin C content was equal to 235.80 mg/100 g DM in the fresh strawberries. These authors noticed that the low content of vitamin C in the strawberries dehydrated in the sucrose solution and juice concentrates could be due to the low content or lack of vitamin C in these osmotic agents.
Table 4
Contents of ascorbic acid, total carotenoids and sugars in fresh orange slices and after their 3-h osmotic dehydration in a sucrose solution and pearly pear molasses.
There was no significant difference in the total carotenoid content of osmotically dehydrated orange slices between sucrose (20.54 mg/kg DM) and molasses (21.53 mg/kg) treatments, while fresh slices boasted a total carotenoid content of 31.44 mg/kg DM (Table 4). Azoubel et al. [2008] found that the total carotenoid content of mango slightly decreased (around 3% of loss) after osmotic dehydration in a sucrose solution for 80 min, and it could be partially associated with pigment diffusion from the fruit to the solution.
In osmotically dehydrated orange slices after 3 h, sugar composition displayed notable differences between treatments in sucrose and molasses solutions (Table 4). A higher content of sucrose was determined in the sucrose-treated slices (41.41 mg/100 g DM) and the molasses-treated slices (38.10 mg/100 g DM) as compared to the fresh slices (2.41 mg/100 g DM). The molasses-treated slices demonstrated higher glucose (5.47 mg/100 g DM) and lower fructose (1.80 mg/100 g DM) contents compared to the sucrose-treated slices (0.77 mg/100 g DM and 3.30 mg/100 g DM, respectively). Kowalska et al. [2023] also reported that the sucrose content increased in strawberry dehydrated in a sucrose solution, while it was reduced after immersion in fruit juice concentrates as compared to fresh strawberry. They explained that the second observations could be due to the sucrose leak from the strawberry tissue to fruit juice concentrates. These authors also found an increase in glucose and fructose contents in strawberries immersed in strawberry and cherry juice concentrates and a decrease in these sugars content in strawberries dehydrated in sucrose and chokeberry juice concentrates. Furthermore, the fructose content was higher in the fruits treated in the strawberry juice concentrate. They deduced that these differences can be result of the different molar masses of the osmotic solutions used. Those with lower molar masses allow for an increased diffusion of the substance into the tissue of the raw material.
CONCLUSIONS
This study showcased the effective OD of orange fruit using both sucrose and prickly pear molasses solutions, each influencing dehydrated fruits quality differently. While both treatments demonstrated distinctive patterns in total mass loss, water loss, solid gain, and the ratio of water loss to solid gain, color, texture and microstructure. Notably, no significant difference was found in water activity between orange slices dehydrated in the sucrose solution and prickly pear molasses. Dehydration in molasses resulted in higher antioxidant capacity of orange slices and preservation of ascorbic acid as compared to the sucrose solution. According to the sugar profiles, orange slices dehydrated in molasses had favored glucose content and reduced fructose content compared to the product obtained by immersion in the sucrose solution.
The choice between sucrose solution and prickly pear molasses hinges on desired characteristics, with molasses offering faster dehydration, enhanced antioxidant activity, and a softer texture, offset by deeper color changes and higher sugar absorption. Conversely, dehydration in a sucrose solution yields a lighter, firmer product with a more balanced sugar profile, albeit with slower dehydration and reduced antioxidant properties. This study paves the way for faster, healthier orange dehydration. By optimizing molasses use or finding new natural agents, we can create products with diverse colors, textures, and nutrient profiles to suit different consumer tastes.