INTRODUCTION
Mango (Mangifera indica L.) is one of the most widely consumed tropical fruits due to its high nutritional and economic values [Occena-Po, 2006]. It has been found to contain numerous bioactive phytochemicals, including phenolics, terpenoids, carotenoids, and phytosterols with antioxidant, anti-inflammatory, immunomodulatory, antibacterial, anti-diabetic, anti-cancer, and anti-microbial benefits [Mirza et al., 2021]. However, mango fruits are extremely perishable and delicate; thus special care must be taken during their transport and storage. Mangoes are a very marketable commodity, and the processing sector can increase its value by transforming them into frozen goods, pulp, and powders among which mango powder holds a lot of promise as a nutritional supplement because of its high nutritional value [Owino & Ambuko, 2021]. Nevertheless, the production of mango powder faces many difficulties because the high sugar content of the fruits hinders moisture removal to reach the final desired moisture content, whereas the stickiness and clumping lessen the recovery rate of product and make the drying process more challenging to operate [Djantou et al., 2007]. Therefore, the main technical problem is to achieve the final moisture content as well as to prevent the adverse phenomenon of stickiness and clumping that occurs during the drying of mango [Truong et al., 2005]. The stickiness of high-sugar products has been determined to be directly associated with the glass transition temperature (the value at which amorphous polymer chains below the phase transition point shift from the crystalline state to the elastic state, symbolized as Tg, is low) [Roos et al., 1996]. At the same time, dried products are prone to clumping when grinding or during storage [Roos et al., 1996; Slade et al., 1991]. Two methods commonly used to overcome these problems are (i) product formulation, and (ii) process optimization [Djantou et al., 2012]. The powder quality and the drying performance can be enhanced through the addition of additives to reduce the moisture content to an appropriate value [Bhandari et al., 1993].
Mangoes are dried using a wide variety of techniques around the globe; for example, Pott et al. [2005] improved the quality of unsulfided mango slices by drying at high temperature (80°C) for about 6 h while Wang et al. [2018] evaluated the thermal efficiency of the indirect forced convection solar drying and analyzed the drying kinetics of mango. Furthermore, Caparino et al. [2012] investigated the physical and microstructural properties of mango powder affected by different drying processes and concluded that Refractance Window® (RW) drying produced mango powder that was on par with that of the freeze-dried powder and even higher in quality than the spray-dried one. The same conclusion in terms of the drying efficiency of the RW method was verified in the study by Zotarelli et al. [2017]. Spray-drying technique is considered a suitable technique in the production of fruit powders thanks to its short drying time, which effectively reduces the loss caused by the decomposition of chemical compounds in the product along with the product moisture of 2–5 g/100 g, water activity of 0.2–0.6, and increased storage stability [Marques et al., 2007; Tan et al., 2011]. The application of the RW drying technique to mango pulp resulted in the production of high-quality mango powder, thereby demonstrating the effectiveness of the high heat drying technique in removing water from mango pulp and overcoming the stickiness that is the primary issue with spray drying [Nguyen et al., 2022a,b].
A new drying technology of the fourth generation, such as infrared (IR) drying, can be applied to the drying of mango pulp, particularly thin layers with high contact surfaces [Allanic et al., 2017]. The emitted radiation is of a narrow wavelength and the heat transfer efficiency is between 80% and 90% [Sadin et al., 2014]. Uniform heating, reduced processing time, increased heat transfer and energy absorption rate, and a better end product are some of the benefits of using IR drying [Zhu & Pan, 2009]. For instance, the IR approach accelerated the drying process of apples by 50% as compared to the convection heat method [Nowak & Lewicki, 2004]. Therefore, the combination of IR and hot air provides a synergistic effect, which leads to a more efficient drying process [Afzal et al., 1999; Nawirska et al., 2009]. The application of infrared drying to mango sections has been the subject of research [Doymaz, 2017; Yao et al., 2020]. Doymaz [2017] reported that the final moisture content of dried mango slices was around 0.15 g water/g dry weight (DW), which corresponds to 13 g/100 g on a wet basis. Another investigation conducted by Yao et al. [2020] exposed a moisture content of about 0.20 g water/g DW, which corresponds to 16.7 g/100 g on a wet basis. These moisture contents were considered inappropriate for the grinding process performed in the production of fruit powder. At this time, empirical evidence regarding the drying of mango pulp via infrared is lacking. However, the fruit powder manufacturing sector shows great potential for implementing infrared drying technology, owing to its easy scalability and remarkable energy efficiency. Based on the favorable findings observed in the production of mango powder via RW drying of mango pulp, it is believable to suggest that infrared drying could exhibit remarkable efficacy when applied to mango powder. Thus, the purpose of this study was to assess the impact of IR drying conditions on drying characteristics and quality of resulting mango powder from mango pulp. These experimental results will contribute to the improvement in production towards the best quality and lowest production cost.
MATERIALS AND METHODS
Material and its pretreatment
Mature and evenly ripe mango (Mangifera indica L.) fruits were collected in Thanh Phuoc hamlet (Thanh Phong commune, Thanh Phu district, Ben Tre province, Vietnam) with soft texture and characteristic aroma. After being washed, peeled and sliced, mango slices were steamed for 3 min and then cooled immediately in cold water, followed by grinding in a commercial blender and sieving through 16-mesh sieves. Afterward, distilled water was used to adjust the mango pulp to 11 and 16°Brix. Mango pulp was subsequently sealed in polypropylene bags and frozen at –18°C until it could be employed again.
Chemicals
Chemicals used in the study were purchased from Sigma-Aldrich (Singapore), including 2,2-diphenyl-1-picrylhydrazyl (DPPH) radical (purity 99%), 6-hydroxy-2,5,7,8-tetramethylchroman-2-carboxylic acid (Trolox, purity 99%), gallic acid (purity 99%), 3,5-dinitrosalicylic acid (DNS, purity 99%), and Folin-Ciocalteu reagent (FCR). Maltodextrin (dextrose equivalent of 16–20), used as a carrier, was purchased from Merck Millipore (Burlington, MA, USA).
Experimental design and infrared drying operation
In this study, an infrared drying system was self-fabricated in a laboratory setting which has previously been effectively applied to drying avocado powder [Nguyen et al., 2021]. The drying system consisted of a drying chamber (length × width × height of 64×23×27 cm) equipped with an infrared radiation module (ITT 1000W 235V-01X0, maximum capacity of 1,000 W, Germany) placed 20 cm away from the surface of drying materials. Infrared radiation was controlled through a thermometer with an accuracy of 0.5°C and a delay of 0.5 s. The three-factor full factorial design including total soluble solids (11 and 16°Brix), drying temperature (70, 75, and 80°C), and maltodextrin content (0, 6, and 9 g/100 g pulp) was used. The moisture content of the dried samples was measured at 5-min intervals by an MB23 moisture analyzer (Ohaus, Parsippany, NJ, USA) for which the drying was discontinued at the moisture content of 0.04 g/g DW. The mathematical model and kinetic parameters for the infrared drying of mango pulp were then determined based on the experimental data of the moisture content change during drying. Besides, retention of total phenolic content (TPC), retention of reducing sugars (RS), total titratable acidity (TA) and retention of DPPH radical scavenging activity of mango powder were used to analyze the influence of factors on the infrared drying of mango pulp.
Mathematical models for thin-layer drying curves
During prolonged drying, for the moisture content at equilibrium state is comparatively small compared to that at different time intervals (Mt, g water/g DW) or the initial value (M0, g water/g DW), the moisture ratio (MR) should be determined according Equation (1) [Pala et al., 1996]:
Many mathematical models have been proposed to predict how foods will dry [Onwude et al., 2016]. This study fitted drying curves to the semi-theoretical and empirical models.
Newton (Lewis) model (Equation 2): The simplest model to describe the drying characteristics of some agricultural products with assumptions that the drying material is arranged sufficiently thin, the air velocity is high, and temperature and relative humidity are constant during drying.
Page model (Equation 3): The experimental model evolved from the Newton model to reduce the error by adding a dimensionless constant (n).
Henderson and Pabis model (Equation 4): The model was derived from the initial term in the general solution of the second Fick diffusion law, operating under the assumption that the general solution approaches being equal to the initial term when the drying process continues for an extended period of time.
Logarithmic model (Equation 5): The model transformed from the Henderson and Pabis model by adding the empirical constant (c).
Midilli model (Equation 6): The model transformed from the Henderson and Pabis model by combining exponential and linear terms.
Weibull model (Equation 7): Experimental model, developed from experimental data.
The coefficient of determination (R2) and the root mean square error (RMSE) were calculated to determine the power of the tested models according Equation (8) and Equation (9), respectively.
where, Mexp,i and Mpre,i are the experimental and predicted moisture ratio, MRexp is the mean value of the experimental dimensionless moisture ratio, and N is the number of observations.Determination of the effective moisture diffusivity
Fick’s second law of diffusion was applied to the mango pulp, which was regarded as an infinite flat plate, in order to determine the movement of moisture. The equation for diffusion is given below (Equation 10):
where, M is the moisture content (g water/g DW), Deff is the effective moisture diffusivity (m2/s), and t is time (s).Crank [1975] provided a general analytical solution for the thin-layer drying under certain conditions, including but not limited to: homogeneous and isotropic product sizes; constant product characteristics; minimal shrinkage; minimal external resistance to heat and mass transfer; sole surface evaporation; uniform initial moisture distribution; constant moisture diffusivity. The answer was as follows (Equation 11):
where, MR is the dimensionless moisture ratio, L is the thickness (m), n is the term in series expansion, and t is time (s).The initial term in the previous series expansion (where n=0) was regarded as an approximation for extended drying periods (Equation 12):
The effective moisture diffusivity was calculated using the non-linear least square method based on the Levenberg-Marquardt method [Marquardt, 1963].
Chemical analysis
Preparation of analytical solution
To prepare the analytical solutions for total phenolic content and antioxidant activity determinations, 0.5 g of powder was extracted with 10 mL of 60% (v/v) methanol under sonication (40 KHz, 240 W, 5 min) in the Pro 100-40D ultrasonic cleaner (Asonic, Ljubljana, Slovenia), followed by being cooled for 20 min at 10°C and centrifuged (1,220×g, 10 min) in the PLC-05 centrifuge (Gemmy Industrial Corp., Taipei, Taiwan). The extraction was then repeated and the supernatant was finally collected for analysis. The analytical samples for the determination of reducing sugar content and total acidity were prepared following the same procedure using distilled water as a solvent.
Total phenolic content determination
Total phenolic content was determined following the International Organization for Standardization (ISO) 14502-1:2005 method [ISO, 2005] which is based on the chromophore formed between phenolics and FCR under alkaline conditions. Briefly, aliquots (0.6 mL) were mixed successively with 1.5 mL of FCR and 1.2 mL of 7.5% Na2CO3 before the absorbance measurement at 765 nm using the UV-9000 spectrometer (Metash, Shanghai, China). Total phenolic content, which was calculated as mg of gallic acid equivalent per g of dried powder (mg GAE/g DW) using the gallic acid standard curve, was used to further estimate its retention (TPC Ret) during drying according to Equation (13).
where:CTPC, before drying and CTPC, after drying were the TPC in the sample before drying and after drying, respectively.Antioxidant activity determination – DPPH assay
DPPH radical scavenging activity was evaluated according to the procedure decribed by Brand-Williams et al. [1995] with minor changes, which is based on the reduction in violet color caused by the reaction of antioxidants and DPPH radical. Briefly, sample aliquots (150 µL) were reacted with 2,850 µL of a working DPPH radical solution (absorbance of 1.10±0.02 at 515 nm) for 30 min in the darkness before being measured for absorbance at the same wavelength. DPPH radical scavenging activity (RSA), which was calculated as mg Trolox equivalent per g of dried powder (mg TE/g DW) using the Trolox standard curve, was used to further estimate its retention (RSA Ret) during drying according to Equation (14).
where: CRSA, before drying and CRSA, after drying were the DPPH radical scavenging activity of the sample before drying and after drying, respectively.Reducing sugar content determination
The reducing sugar (RS) content was determined according to DNS assay [Miller, 1959] by mixing 1 mL of samples with 1 mL of DNS reagent under boiling conditions for 10 min. After sample cooling to ambient temperature, distilled water (2 mL) was added, and absorbance was measured at 540 nm to estimate the RS content and its retention (RS Ret) during drying from Equation (15).
where: CRS, before drying and CRS, after drying were the reducing sugar of the sample before drying and after drying, respectively.Total titratable acidity determination
The extract (10 mL) was titrated with standard NaOH solution in the presence of phenolphthalein as an indicator. The titration stopped when the solution attained a pale pink color that persisted for 30 s. Total titratable acidity (TA) was calculated by Equation (16).
where: VNaOH and Vsample were the volume of titrated NaOH solution and extract sample, respectivley.Optimization method
The study employed a numerical optimization technique to simultaneously optimize multiple responses using Design Expert version 13.0 (Stat-Ease, Inc., Minneapolis, MN, USA). To address the desire to find a solution that accommodates various responses, the objectives were combined into an overall composite function, denoted as D(x), referred to as the desirability function. This function is defined by Equation (17) [Myers et al., 2016].
where: d1, d2, …, dn are responses and n is the total number of responses in the observations.The function D(x) represents the optimal intervals for each response (di). Desirability is a quantitative measure that varies between zero (indicating the least desirable condition) and one (representing the most desirable condition) at the desired outcome. Numerical optimization identifies the point that maximizes the desirability function [Myers et al., 2016].
Statistical analysis
Experiments were conducted in triplicates, and data were fitted by the non-linear least square method based on the Levenberg-Marquardt method. After one-way analysis of variance (ANOVA), Tukey’s honestly significant difference test was performed. StatPlus software (AnalystSoft, Brandon, FL, USA) was used to analyze the Pearson correlations between variables.
RESULTS AND DISCUSSION
Drying characteristics of mango pulp during infrared drying
The changes in moisture ratio and drying rate under different IR drying conditions of mango pulp are shown in Figures 1 and 2, respectively. As can be seen in Figure 1, drying times can be reduced by increasing drying temperatures. Drying time, however, was proportional to the amount of maltodextrin or total soluble solids in the mango pulp. In the meantime, the lower total soluble solids required a lower amount of maltodextrin, and higher drying temperature led to the higher drying rate. Also, based on the drying rate curve data (Figure 2), infrared drying of mango pulp proceeded primarily in the deceleration stage.
Figure 1
The change of moisture content vs time in infrared drying of mango pulp under different conditions including temperature of 70–80°C, maltrodextrin content of 0–9 g/100 g pulp, and total soluble solids of pulp at 11 and 16°Brix. DW, dry weight.
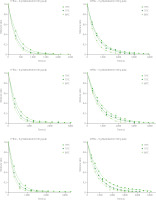
Figure 2
Drying rate curves of infrared drying of mango pulp at different conditions including temperature of 70–80°C, maltrodextrin content of 0–9 g/100 g pulp, and total soluble solids of pulp at 11 and 16°Brix. DW, dry weight.
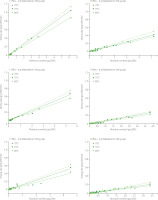
Experimental data on moisture ratio was used to select a model that characterizes the moisture removal in mango pulp during IR drying. The mathematical model was chosen based on its highest coefficient of determination (R2) and the lowest root mean square error (RMSE), all of which were presented as mean values (Table 1). The high R2 values accompanied with the low errors were used to rank the mathematical models in the descending order of Weibull > Midilli > Logarithmic > Page > Henderson and Pabis > Newton models. Apparently, the Weibull model was the best predictive among the models investigated. This conclusion is not in agreement with the report of Onwude et al. [2016], who suggested that the Midilli model was the best to characterize the thin layer drying of fruits and vegetables.
Table 1
Results of non-linear regression analysis of kinetic models of infrared drying of mango pulp and values of effective moisture diffusivity (Deff).
However, the experimental data was not assessed on the Weibull model in these investigations. On the other hand, Midilli and Weibull models have been reported as the most compatible with experimental data in several research, including those looking at the drying of persimmon slices [Doymaz, 2012] and sliced lemongrass [Nguyen et al., 2019] while the latter model can also be utilized to identify the drying kinetics such as convective drying of sliced mango [Corzo et al., 2010], pepino fruit (Solanum muricatum Ait.) [Uribe et al., 2011], quince [Tzempelikos et al., 2015], and longan [Ju et al., 2018]. Therefore, in this study, we confirm that the Weibull model is the most suitable to characterize the moisture removal of mango pulp under IR drying.
The effective moisture diffusivity during the IR drying of mango pulp has been determined and values are presented in Table 1. The correlation between the effective moisture diffusivity and process condition paremeters (R2=0.99, p<0.0001) was described by Equation (18):
where, x1, x2, and x3 correspond to the coding levels of total soluble solids, drying temperature, and maltodextrin content, respectively.It is shown that the lowest effective moisture diffusivity (3.086×10–10 m2/s) was observed at drying conditions of 70°C, 16°Brix, and 9% maltodextrin, whereas 80°C, 11°Brix, and 0% maltodextrin resulted in the highest Deff value of 14.78×10– 10 m2/s. These results were consistent with those reported for thin layer drying of fruits and vegetables [Onwude et al., 2016] and IR drying of tomatoes at 60–80°C (1.094×10–9 to 4.468×10–9 m2/s) [Sadin et al., 2014], carrots at 60–80°C (2.38×10–9 to 10.30×10–9 m2/s) [Wu et al., 2014], bell peppers at 50–80°C (1.75×10–10 to 8.97×10–10 m2/s) [Nasiroglu & Kocabiyik, 2009], anise at 60–80°C (5.022×10–10 to 9.557×10–10 m2/s) [Wen et al., 2020], and blueberries at 60–90°C (2.24×10–10 to 16.4×10–10 m2/s) [Shi et al., 2008]. It is possible to infer that even supposing the same IR drying temperature, the drying of mango pulp showed a greater Deff value than the drying of bell peppers, anise, and blueberries, but a lower value than during drying of tomatoes and carrots.
Effects of infrared drying on the quality of mango powder
The effects of IR drying conditions on the quality of mango powder including the retention of total phenolic content, DPPH radical scavenging activity, reducing sugars and the value of total titratable acidity are presented in Figure 3, 4, 5 and 6, respectively. The results demonstrate that higher temperatures and increased maltodextrin content, together with decreased total soluble solid content, enhanced the retention of TPC, RS, RSA (TPC Ret, RS Ret, and RSA Ret, respectively), and TA. The correlation between the quality indicators of mango powder and process condition parameters were described by Equations (19)–(22).
where, x1, x2, and x3 are corresponding to the coding levels of total soluble solids, drying temperature, and maltodextrin content, respectively.Figure 3
The retention of total phenolic content in mango powder obtained by infrared drying of pulp at different conditions. (A) The results for drying pulp with total soluble solids (TSS) of 11°Brix. (B) The results for drying pulp with TSS of 16°Brix. The same letters above bars of both graphs indicated that the values are not significantly different (p≥0.05).
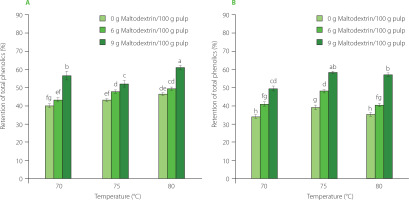
Figure 4
The retention of DPPH radical scavenging activity in mango powder obtained by infrared drying of pulp at different conditions. (A) The results for drying pulp with total soluble solids (TSS) of 11°Brix. (B) The results for drying pulp with TSS of 16°Brix. The same letters above bars of both graphs indicated that the values are not significantly different (p≥0.05).
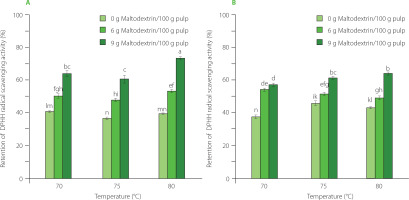
Figure 5
The retention of reducing sugars in mango powder obtained by infrared drying of pulp at different conditions. (A) The results for drying pulp with total soluble solids (TSS) of 11°Brix. (B) The results for drying pulp with TSS of 16°Brix. The same letters above bars of both graphs indicated that the values are not significantly different (p≥0.05).
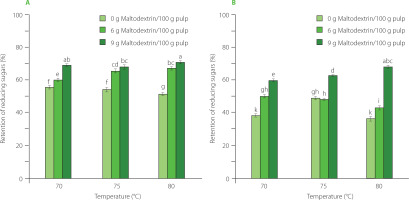
Figure 6
The total titratable acidity in mango powder obtained by infrared drying of pulp at different conditions. (A) The results for drying pulp with total soluble solids (TSS) of 11°Brix. (B) The results for drying pulp with TSS of 16°Brix. The same letters above bars of both graphs indicated that the values are not significantly different (p≥0.05).
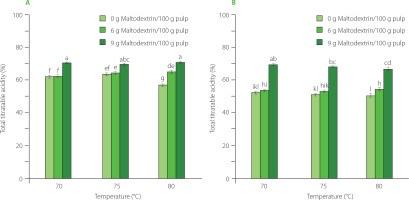
The above regression equations also clearly indicate that the retention of TPC, RS, RSA, and the value of TA were all positively correlated with either the IR temperature or the maltodextrin content.
During thermal processes, the degradation of phenolics is mainly due to the action of enzymes, such as polyphenol oxidase (PPO) and peroxidase (POD) [Tomás-Barberán & Espín, 2001]. For mangoes, PPO and POD were most effective at 50–60°C [Korbel et al., 2013] and were inactivated at high temperatures, i.e. 70–90°C [Queiroz et al., 2008]. According to Sultana et al. [2012], intense heat treatment was able to form new phenolic compounds. During the high-temperature drying, the drying time was shortened, indicating a shorter decomposition reaction time [Nguyen et al., 2020]. It is deduced that a rise in temperature will result in the increased retention of phenolic compounds in mango pulp, which was strengthened by the highest retention of phenolics found in some studies on the high-temperature drying, such as RW drying at 90°C [López et al., 2010] and 95°C [Shende & Datta, 2020] of blueberries and mango pulp, respectively. Regarding the carrier, the increase in maltodextrin content in mango pulp retained phenolics possibly because this carrier hindered the exposure of these bioactives in mango pulp with oxygen, leading to limited degradation [Osorio et al., 2011]. Besides, the addition of maltodextrin to the pulp increased the dry matter, showing high binding capacity to water [Radosta & Schierbaum, 1990], which in turn lowered the water activity and limited the phenolic decomposition. These results are in accordance with findings from other studies on sapodilla (Manilkara zapota) powder [Chong & Wong, 2017] and avocado (Persea Americana Mill.) powder [Nguyen et al., 2023].
Mango is rich in sugars, including reducing sugars such as glucose and fructose [Ribeiro & Schieber, 2010]. During the high-temperature drying, a high-sugar material, like mango, will easily lose reducing sugar content, primarily due to the Maillard reaction [Jaeger et al., 2010]. As a result, the Maillard process accelerated the loss of reducing sugars at higher drying temperatures. However, an increase in the maltodextrin content limits the exposure of the substrates, thereby markedly reducing the extent of sugar losses. Total titratable acidity was shown to be significantly affected by maltodextrin content and drying temperature. Specifically, a greater amount of maltodextrin helped form a barrier to minimize the nutrient loss during heat treatment while the depletion of acidic compounds increased mostly at higher temperatures with increased thermal energy.
The investigation also revealed the positive correlations between the retention of TPC, RS, RSA, and the value of TA as shown in Table 2. The findings indicated that the retention of TPC exhibited significant corellation with the retention of DPPH radical scavenging activity with a correlation coefficient of 0.853 (p<0.01). This implies that the capacity of mango powder to scavenge DPPH radicals displayed a similar pattern to that of the content of phenolic compounds, which are known for their antioxidant properties [Huyut et al., 2017; Sikwese & Duodu, 2007]. Thanks to their specific chemical structure, these molecules can scavenge free radicals and also prevent their further production in the presence of transition metal ions as catalysts [Huyut et al., 2017; Sikwese & Duodu, 2007]. The retention of TPC also exhibited a strong positive connection with the retention of RS, and values of TA (Table 2). The similar trend of changes in TPC, RS, and TA could be inferred in the infrared drying process of mango pulp under the conditions used in the study.
Table 2
Coefficients of Pearson correlations between the retention of DPPH radical scavenging activity (RSA), retention of total phenolic content (TPC), retention of reducing sugars (RS), and the values of total titratable acidity (TA).
Retention of RSA | Retention of TPC | Retention of RS | |
---|---|---|---|
Retention of TPC | 0.853 (p=0) | – | |
Retention of RS | 0.719 (p=9.48×10−10) | 0.856 (p=0) | |
TA | 0.663 (p=4.56×10−8) | 0.819 (p=0) | 0.915 (p=0) |
Optimization of infrared drying for mango pulp
This study applied a numerical optimization technique to simultaneously optimize multiple responses, such as effective moisture diffusivity, DPPH radical scavenging activity retention, TPC retention, RS retention, and TA value. The specific objectives for each factor and response were selected, and distinct weights were given to each objective to adjust the form of the desirability function (Table 3). The contour plot of the desire function for mango pulp under various IR drying settings are displayed in Figure 7. The desirability function reached the highest value of 0.916 (Table 3). The predicted values for all independent and dependent parameters resulting from the optimization process using the desirability function are shown in Table 3. The optimal values of control parameters were 11°Brix, 80°C, and 9% maltodextrin content. Under these specific drying conditions, the mango powder was achieved with the highest retention of TPC (59.874%), retention of RS (71.044%), TA (10.141%), retention of RSA (65.051%), and Deff (14.033×10-10 m2/s).
Table 3
Criteria for optimization of factors and responses and their values of optimal point within the desirability region for infrared drying of mango pulp.
CONCLUSIONS
In this study, infrared drying of mango pulp under different conditions was performed. The Weibull model was useful for describing the decline in mango pulp’s moisture content during infrared drying. The effectiveness of infrared drying of mango pulp was greatly influenced by drying temperature, total soluble solid content, and maltodextrin content. Temperature had a positive effect on water removal while total soluble solid content and maltodextrin showed the opposite effect. Moreover, total phenolics and DPPH radical scavenging activity were better retained with increasing IR temperature (from 70 to 80°C) or maltodextrin content (from 0 to 9 g/100 g pulp). Therefore, it is important to set adequate pretreatment conditions or employ additives to maintain powder’s quality in order to take advantage of the infrared drying. Finally, the findings indicated that the implementation of infrared drying of mango pulp with 11°Brix and 9% maltodextrin content at 80°C would ensure the highest quality of mango powder.